NatureScot Research Report 1326 - Scottish Blue Carbon - a literature review of the current evidence for Scotland’s blue carbon habitats
Year of publication: 2023
Authors: Cunningham, C. and Hunt, C.
Cite as: Cunningham, C. and Hunt, C. 2023. Scottish Blue Carbon - a literature review of the current evidence for Scotland’s blue carbon habitats. NatureScot Research Report 1326.
Keywords
blue carbon; carbon stocks; sequestration; production; climate change mitigation; adaptation; nature-based solution; organic; inorganic; marine habitats
Background
Blue carbon refers to carbon captured and stored in the coastal and marine environment. The ocean plays a critical role in climate regulation by naturally absorbing excess carbon dioxide (CO2) from the atmosphere. Blue carbon habitats contribute to climate regulation because they can be extremely efficient at converting CO2 into organic (biological) material and storing it within living biomass, root systems, and sediments. Globally, three specific blue carbon habitats - mangroves, saltmarshes, and seagrasses - make up less than 0.5% of the total seafloor area. However, they are estimated to sequester ten times more CO2 annually than the same area of terrestrial forest and contribute an estimated 50% of the total burial of organic carbon in ocean sediments. Other coastal and marine habitats also contribute to carbon cycling, sequestration, and storage, including biogenic calcifying reefs, kelp, and vegetated sand dunes, although less is currently known about these systems and the long-term fate of carbon they trap or store.
Climate change will alter processes of climate regulation, potentially affecting carbon sequestration and storage potential within blue carbon habitats. The impact of climate change on the ocean has been recognised internationally, and as a result, policy commitments for ocean protection and restoration are increasing. Blue carbon contributions towards climate change mitigation are also being acknowledged within national carbon accounts and greenhouse gas inventories. However, blue carbon habitats are also directly threatened by human activities that cause their degradation and destruction. This damage can result in the release of long-term stored carbon as emissions and an associated loss of future sequestration potential. Blue carbon habitats are essential for biodiversity and some habitats are designated features of Marine Protected Areas (MPAs) and classified as Priority Marine Features (PMFs) within Scotland. Blue carbon habitats can also provide a range of ecosystem services including the provision of nursery habitat for commercial fish species, water filtration, and flood protection.
For these reasons, it is essential to understand the extent and distribution of blue carbon habitats, their potential for carbon sequestration and storage, and the threats posed to them. This will enable evidence-based decisions and development of policies for effective protection, restoration and management that will secure the health of blue carbon habitats and the ecosystem and societal benefits they can provide.
This literature review was compiled with the aim of consolidating currently available information for Scottish blue carbon habitats. It builds upon the first Scottish national inventory for blue carbon stocks, which was undertaken in 2014. Information is presented on the distribution and extent, estimated carbon standing stock and carbon sequestration capacity, threats, and blue carbon research needs associated with the marine and coastal habitats considered. Initial assessments of data confidence are included and key evidence gaps identified for future research.
Main findings
The main findings pertaining to current knowledge about carbon storage and sequestration in coastal and marine habitats are summarised below. Organic carbon (OC) and inorganic carbon (IC) values have been differentiated and included where data are available.
Blue Carbon Stock and Sequestration Values
- Scottish blue carbon habitats are wide ranging and include: vegetated coastal habitats (saltmarsh, sand dunes, and machair) and nearshore habitats (including intertidal and subtidal seagrass beds and kelp) that produce and store organic carbon; calcifying aggregations (e.g., maerl, serpulid aggregations, and native oysters) that produce and store inorganic carbon within their structures; and seabed sediments, which receive, bury, and store organic and inorganic carbon.
- Scottish blue carbon habitats make a significant contribution to carbon sequestration and storage, both for organic and inorganic carbon. The distinction between forms of carbon is important when assessing carbon stocks and for developing enhancement and protective measures.
- The sequestration of CO2 into biomass and its subsequent burial as organic carbon in sediments is a carbon sink process and contributes to climate change mitigation. In contrast, the process of calcification by calcifying aggregations results in a net release of CO2. As a result of this calcification process, inorganic carbon stores do not contribute to climate change mitigation. However, calcifying aggregations are essential components of a healthy marine ecosystem and function as long term stores of inorganic carbon.
- Seabed sediments (fjords, coastal, and shelf) in the Scottish sector of the UK Exclusive Economic Zone (EEZ) hold the majority of the carbon stock. An estimated 357 (± 72.2) Mt organic carbon and 2,265 (± 156.3) Mt inorganic carbon totalling 2,622 Mt C is held within the top 10 cm of seabed sediments. The total value will be significantly higher accounting for the greater than 10 cm thickness of seabed sediments.
- The total annual sequestration capacity for Scotland’s seabed sediments is currently unknown due to a lack of available data to constrain burial rate estimates across large areas of the shelf. However, Scottish fjordic sediments are unique hotspots for sedimentary carbon burial, with estimated sequestration rates of 57.1 (± 10.9) g OC m-2 yr-1, burying an estimated 84,000 t OC yr-1 (308,000 t CO2-eq). These rates are likely to be higher than sequestration rates for the wider shelf sea sediments. However, the total sequestration capacity of all seabed sediments will be significantly higher.
- The cumulative carbon standing stock of Scottish blue carbon habitats (excluding sediments) is estimated to be ~5.34 Mt C. This includes an estimated organic contribution of 3.08 Mt OC and an estimated inorganic contribution of 2.26 Mt IC.
- This review estimates that Scottish vegetated coastal and nearshore habitats, excluding kelp, sequester a combined 18,495 t OC yr-1 (~0.02 Mt C yr-1 or 68,000 t CO2-eq). In comparison, kelp is estimated to produce approximately 1.4 Mt OC yr-1.
- Scottish saltmarshes have an estimated standing stock of 1.15 ± 0.25 Mt OC (~ 50% of the coastal OC stock) and an average sequestration rate of 87.45 g OC m-2 yr-1, determined from representative mud-rich and sand-rich saltmarsh sites across Scotland, accumulating approximately 4,385 ± 481 t OC annually. The rate varies between saltmarsh zone, with the lower zone generally observed to have higher sequestration rates attributed to more frequent tidal inundation.
- Sand dune habitat within Scotland has an estimated organic carbon stock of 0.75 Mt and a total carbon sequestration capacity of ~9,000 t OC yr-1, although this estimate is based on the only carbon sequestration rates currently available, that are derived from a Welsh dune system.
- Seagrass beds within Scotland have an estimated standing stock of 0.17 Mt OC and a sequestration capacity of 1,021 t OC yr-1. These values are expected to be under-estimated due to likely unmapped areas of seagrass across Scotland.
- Kelp is estimated to store ~0.6 Mt OC as standing stock within living biomass. Kelp has a significant production capacity of 1.4 Mt OC yr-1 and an average production rate of 378 g OC m-2 yr-1,based on values from Scotland. This carbon is not sequestered locally, with kelp acting as a blue carbon donor habitat instead.
- Habitats grouped under calcifying aggregations are estimated to hold a combined 2.26 Mt IC and have a total inorganic carbon production capacity of 2,519 t IC yr-1, although many of the calcifying habitats have unknown extent values and IC productions rates and thus there is large uncertainty with these estimates.
- Maerl deposits within Scotland are estimated to have a standing stock of 1.96 Mt IC; this is based on an average bed thickness of 0.6 m and a likely underestimated national extent.
- Serpulid aggregations could be one of the most productive carbonate habitats with an estimated inorganic carbon production rate of 420 g IC m-2 yr-1, however there are large uncertainties with this value. Contributions of this habitat to IC stocks are relatively low due to limited habitat extent in Scotland, which is also observed to be declining.
Threats and Management Opportunities
- Climate change threatens Scotland's blue carbon habitats, in particular the impacts of ocean acidification, warming sea temperatures, changes in rainfall, and increased storminess.
- Coastal development and competing land-use have led to the loss of blue carbon habitats, in particular saltmarsh, sand dunes, and seagrass.
- Bottom-contacting fishing gear is considered one of the largest threats to marine blue carbon habitats, including organic-rich sediments, however the science relating to the fate of OC in disturbed sediment is still limited.
- Managing pressures that have known potential to degrade blue carbon habitats, for instance high nutrient levels, invasive species, and trampling impacts from the likes of livestock and coastal recreationists, can increase carbon sequestration and storage potential.
- The impact on blue carbon services by some manageable activities such as grazing is still unclear, presenting an important research gap to inform management.
Conclusions and Recommendations
- Scottish blue carbon habitats make a significant contribution to carbon sequestration and storage as organic and inorganic carbon stores. However, when considering opportunities for climate change mitigation, only organic carbon stores should be considered.
- A lack of extent data for many of Scotland's blue carbon habitats impedes the calculation of accurate estimates of carbon stocks. Additionally, there is a paucity of Scottish-specific sequestration and burial rate data for these habitats.
- Additional data would provide opportunities for improved blue carbon accounting and spatial management options to maintain and/or enhance blue carbon stocks.
- Protection of these habitats increases the opportunities for CO2 sequestration and long-term carbon storage.
- Blue carbon is a component of the overall contribution that nature makes to Scotland’s Blue Economy asset base. The relative contribution of blue carbon should be considered in tandem with other ecosystem functions and services. All of the habitats reviewed provide a wealth of other benefits, from coastal defence to supporting biodiversity, and any management should take into account all aspects of ecosystem function.
Figures 1-3 are infographics that provide a visual summary of the main blue carbon stock and sequestration values.
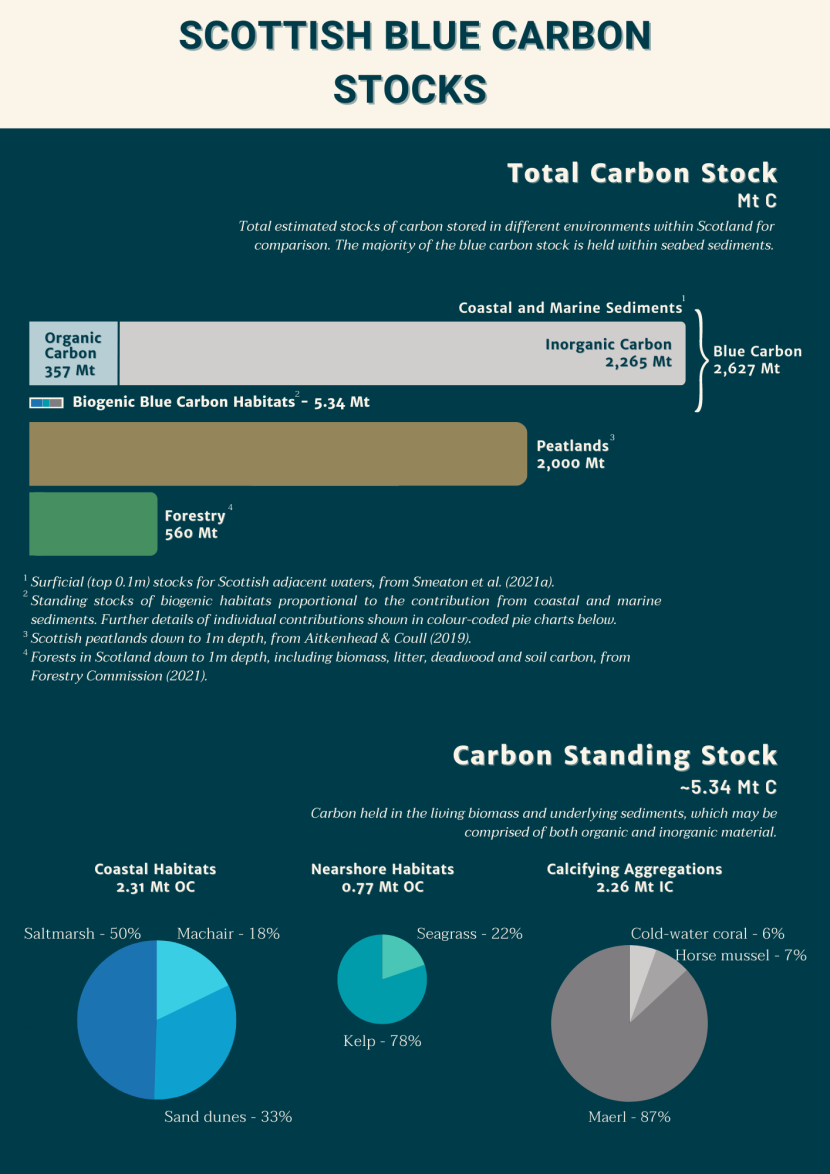
In the top half of the figure, the total carbon stocks are presented in bar chart format. There are three bars consisting of total carbon stock values in Scotland’s blue carbon (light blue and beige bar), peatlands (brown bar) and forests (green bar). The contribution from sediment is split into organic carbon (light blue) and inorganic carbon (beige). An additional small bar is scaled relative to the stock contribution that the biogenic blue carbon habitats make relative to the size of the sedimentary contribution. It consists of three small squares to represent coastal habitats (blue), nearshore habitats (green) and calcifying aggregations (light brown).
In the bottom half of the figure, there are three pie charts presenting the total standing stock estimates for the biogenic habitats at a larger scale than the small bar chart in the top half of the figure. This is carbon held in living biomass and underlying sediments. The blue shaded pie chart represents the individual contributions made from coastal habitats (saltmarsh – 50%; Machair – 18%, and sand dunes 33%). The green shaded pie chart represents the individual contributions made from nearshore habitats (seagrass – 22%; kelp 78%). The beige shaded pie chart represents the individual known contributions made from calcifying aggregations (cold-water coral 6%; horse mussel – 7%, and maerl 87%).
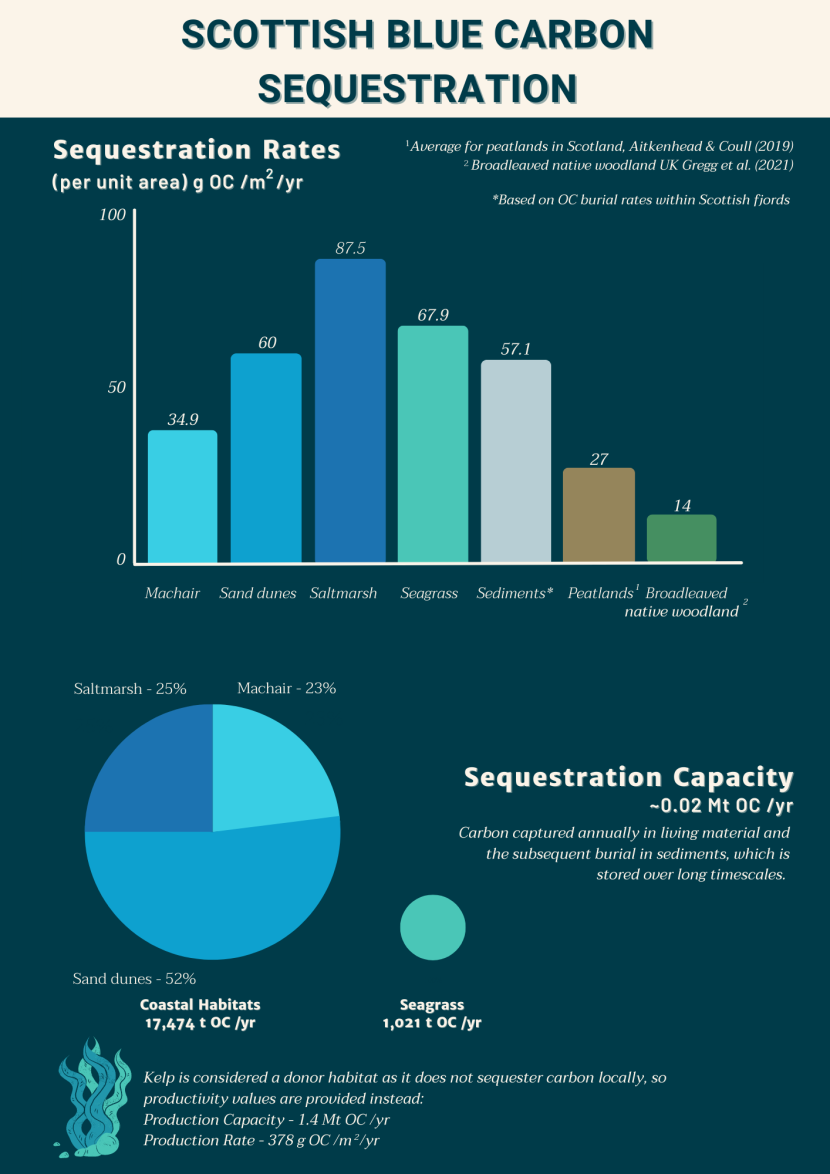
The top half of the infographic consists of a bar chart showing the individual sequestration rates for blue carbon habitats (where the literature is available) and for peatland and forest habitat as a comparison. The bars are coloured individually to stand out. Machair is light blue; sand dunes are a medium blue; saltmarsh is a dark blue; seagrass is a medium green; sediment is beige; peatland is brown; forest is dark green. Blue carbon habitats have higher sequestration rates than terrestrial habitats.
The bottom half of the infographic consists of pie charts showing the relative sequestration capacity of blue carbon habitats as estimated within this review. This is the carbon captured annually by these habitats. The coastal habitat pie chart is in shades of blue and is made up of saltmarsh (25%), machair (23%) and sand dunes (52%). A green pie chart represents the total sequestration capacity of seagrass, which is approximately 17 times smaller than the combined coastal habitats. Finally, the values for the production capacity and rate are provided next to a cartoon image of kelp.
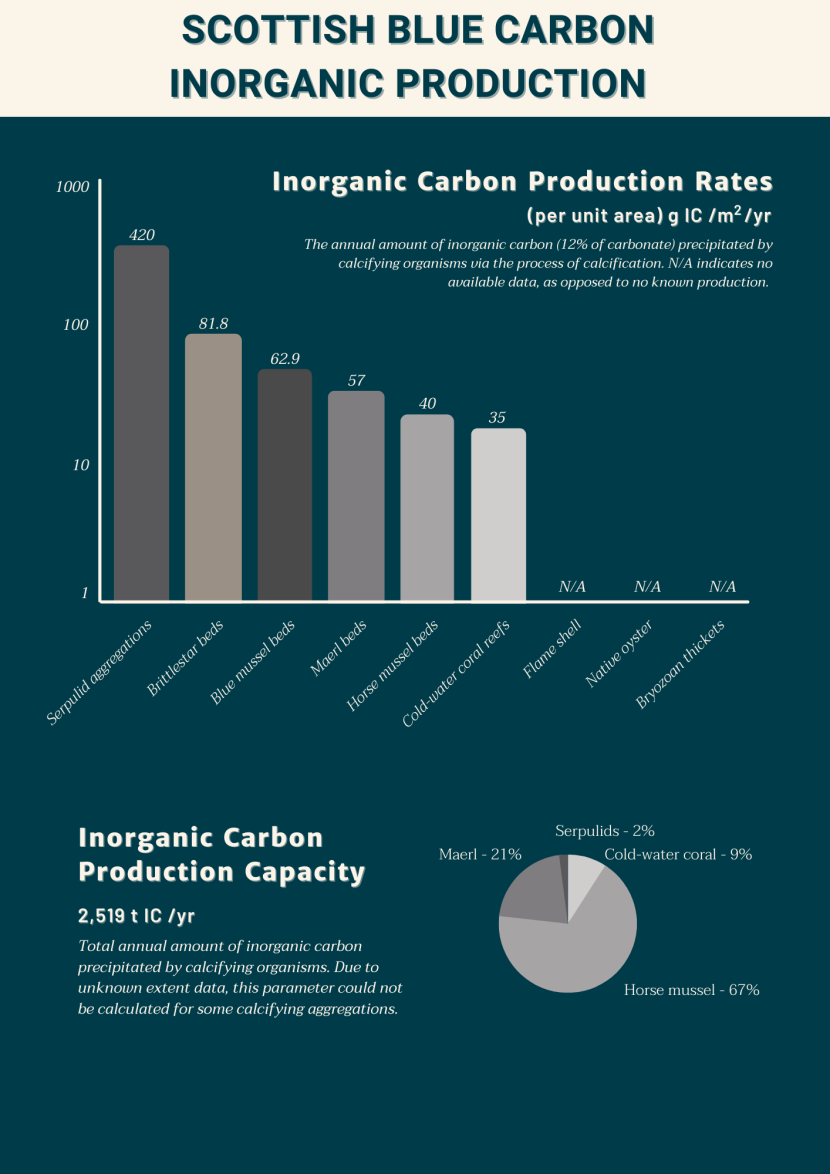
The top half of the infographic consists of a bar chart in shades of brown and beige showing the individual inorganic carbon production rates for calcifying aggregations (where the literature is available). Serpulid aggregations has the highest sequestration capacity (420 g IC / m2/ yr) and cold-water coral reefs are estimated to have the lowest sequestration capacity (35 g IC / m2/ yr). N/A represents no available data for flame shell, native oyster and bryozoan thicket.
The bottom half of the infographic consists of a pie chart showing the relative inorganic carbon production capacity of calcifying aggregations as estimated within this review. This is the amount of inorganic carbon produced annually by these habitats. The pie chart is in shades of brown and is made up of serpulids (2%), cold-water coral (9%), horse mussel (67%), and maerl (21%).
Acknowledgements
We would like to thank the Scottish Blue Carbon Forum for funding and supporting Caitlin Cunningham during her placement at NatureScot to carry out this review.
The authors would like to thank all members of the NatureScot Sustainable Coasts and Seas, and Marine Ecosystems teams, who have provided their time and expertise to review and input into this document. Special thanks to Tracey Begg, Cathy Tilbrook, Katie Gillham, Sarah Cunningham, Ben James, Flora Kent, Nick Everett, Stewart Angus, and Kelly James of NatureScot, and Bill Turrell of the Marine Scotland Directorate, also a member of Scottish Blue Carbon Forum Steering Committee.
Abbreviations
Calcium carbonate (CaCO3)
Carbon (C)
Carbon Hydrogen Nitrogen (CHN)
Carbon dioxide (CO2)
Exclusive Economic Zone (EEZ)
Greenhouse gas (GHG)
Inorganic carbon (IC)
Invasive non-native species (INNS)
Loss on ignition (LOI)
Marine Nature Conservation Review (MNCR)
Marine Protected Area (MPA)
Organic carbon (OC)
Organic carbon accumulation rate (OCAR)
Priority Marine Feature (PMF)
Relative Sea Level Rise (RSLR)
Scotland’s Marine Assessment (SMA)
Special Area of Conservation (SAC)
1. Introduction
1.1 Scottish Blue Carbon Evidence
This literature review was compiled with the aim of consolidating currently available information on Scottish blue carbon habitats. It builds upon the first Scottish national inventory for blue carbon stocks, which was undertaken by Burrows et al. (2014) almost 10 years ago. Burrows et al.'s (2014) formative review identified multiple marine habitats that contribute to directly sequestering carbon and/or storing it, either as organic or inorganic forms. At the time, the review highlighted a scarcity of Scottish-specific evidence and research in this area and often resorted to using data values collected outside of a Scottish and wider UK context. Several reports have since been published that have attempted to collate the evidence for blue carbon stocks in various contexts and scales. These include, blue carbon contained within Scottish Marine Protected Areas (Burrows et al., 2017); other national ‘inventories’ such as for England (Gregg et al., 2021), Wales (Armstrong et al., 2021) and Jersey (Chambers et al., 2022a); or for specific habitats at a UK-wide scale (Smeaton et al., 2021a, 2021b). Within this review the evidence is primarily focussed on habitat extent, blue carbon contributions, threats, restoration efforts and key areas of future research.
The results of this literature review will feed into a Scottish Blue Carbon Evidence Map, which is intended to critically evaluate the evidence base identified within this review for its context and rigour. An evidence review is a key mechanism for understanding nuances of the literature and for communicating complex evidence to policy-makers. It will also be used to help prioritise addressing the evidence gaps identified based on a risk assessment.
1.2 Blue carbon overview
The concept “blue carbon” was first introduced to highlight that in addition to terrestrial forests, coastal and marine ecosystems also sequester carbon (Nellemann et al., 2009). It is similar to the term “green carbon”, which refers to the carbon stored in natural ecosystems or the biosphere, although this typically has a terrestrial focus. The definition of blue carbon is still debated, due to differing interpretations relating to valuing the blue carbon resources. This ranges from their importance in the marine carbon cycle, fluxes with atmospheric and terrestrial cycles (Nellemann et al., 2009) and ongoing uncertainties about the fate and longevity of carbon within these systems, to more policy aligned approaches, which aim to quantify the carbon sequestration and storage services these habitats provide (Burrows et al., 2014; 2017; Porter et al., 2020). This review adopts the following broad definition of blue carbon from the Scottish Blue Carbon Forum (2022):
Blue carbon is the carbon captured and stored in marine and coastal ecosystems that accumulates over long timescales through natural processes. In Scotland, blue carbon habitats include saltmarshes, seagrasses, kelp beds, biogenic reefs and geological sedimentary stores, such as seafloor and sea loch sediments.
It includes ‘autochthonous’ carbon captured directly by all biological metabolic process (e.g. photosynthesis and calcification) and ‘allochthonous’ material derived from other sources (e.g. terrigenous) that is subsequently deposited and stored as either organic or inorganic carbon in marine sediments.
1.3 Blue carbon habitats
A lack of consensus exists for which habitats are considered to capture and store blue carbon, with three vegetated coastal systems – mangroves, saltmarshes and seagrasses – more widely accepted in policy and the literature (IPCC, 2019; McLeod et al., 2011). These photosynthetic ecosystems are considered more efficient at sequestering carbon than most terrestrial forests, when normalised for area (McLeod et al., 2011). Tidal inundation of these habitats is highly effective in trapping organic matter, which can be buried in associated, often water-logged, sediments. In the marine environment, calcifying organisms also store inorganic carbon, with coralline algae having an estimated global carbon burial of 1.6 billion tonnes annually (van der Heijden and Kamenos, 2015). The calcifying organisms considered in this review either build tubes, shells or skeletons from calcium carbonate (CaCO3), which may persist on the seafloor or become incorporated into bottom sediments as shell material (Burrows et al., 2014). Biogenic reef habitats can also trap associated biota and sediments within their matrix-like structures, potentially contributing to long term organic carbon storage (Lee et al., 2021). Additionally, macroalgae ecosystems, including kelp beds/forests, have an important role in carbon sequestration through their ability to act as carbon donors to other habitats (Trevathan-Tackett et al., 2015; Krause-Jensen and Duarte, 2016). Coastal and marine seabed sediments receive and accumulate carbon and have a significant role as long term carbon stores.(Smith et al., 2015). This literature review therefore discusses the following Scottish blue carbon habitats:
- Vegetated Coastal habitats –
- Machair
- Sand dunes
- Saltmarsh
- Nearshore habitats –
- Seagrass beds
- Kelp beds
- Calcifying aggregations –
- Maerl beds
- Cold-water coral reefs (Lophelia pertusa)
- Serpulid aggregations (Serpula vermicularis)
- Flame shell beds (Limaria hians)
- Horse mussel beds (Modiolus modiolus)
- Blue mussel beds (Mytilus edulis)
- Native oyster (Ostrea edulis)
- Brittlestar beds (Ophiothrix fragilis)
- Bryozoan thickets (Flustra foliacea)
- Seabed sediments
Whilst there is extensive literature on the role terrestrial habitats have in sequestering carbon (IPCC, 2019), blue carbon is an emerging area of research with prominent gaps in understanding (Macreadie et al., 2019). Research effort differs across habitats and regions, with large uncertainties mostly associated with poor habitat extent estimates and the lack of Scottish specific carbon sequestration and storage rates. As a result, there are various limitations associated with the data presented and these limitations are explored as part of this review.
1.4 Blue carbon terms
Describing the dynamics of carbon cycling is complex and requires clarity of terminology. For the purposes of this review, the following blue carbon definitions are used –
- Standing stock/biological carbon: the carbon held in biological material, which may be comprised of both organic and inorganic material in living biomass.
- Stock: stores of carbon in sediments.
- Geological carbon: the carbon stored through prior sequestration in the underlying sediments of biological habitats or on the seabed.
- Organic carbon (OC): the carbon found in living plants and animals, in organic rich detritus, as dissolved organic carbon, and as particulate organic carbon.
- Inorganic carbon (IC): the carbon dissolved in seawater that forms carbonates, dissolved inorganic carbon and the calcareous skeletons, and hard shell material, formed by living organisms otherwise referred to as particulate inorganic carbon. In blue carbon literature, calcium carbonate (CaCO3) is considered inorganic carbon, with the terms often used interchangeably (Macreadie et al., 2017).
- Carbon sequestration rate: the conversion of carbon dioxide to organic carbon through capture in living material and subsequent burial in sediments, which is stored over long timescales. Long term stores of inorganic carbon may also be considered sequestered, however are dealt with separately under ‘inorganic carbon production rate’.
- Carbon production rate: the annual amount of organic carbon captured in biomass. Net production refers to the annual amount of carbon captured, accounting for losses through respiration.
- Inorganic carbon production rate: the annual amount of inorganic carbon as a percentage of carbonate that is precipitated by calcifying organisms via the process of calcification. During this process, for every mole of carbonate precipitated, 0.63 moles of CO2 are produced. Inorganic carbon constitutes 12% of the carbonate molecule.
1.5 Blue carbon parameters and units
The associated calculations and base units adopted for these parameters are summarised in Table 1. Each habitat section considered uses estimates of extent (ha) and the carbon content by area or volume derived from the literature, to calculate the carbon currently stored (t C) in each habitat. Storage values are presented as area-specific stock estimates (t C ha-1), which can be multiplied by extents of habitats for an overall carbon store (t C). Area-specific standing stock is otherwise known as the carbon density – i.e. carbon stock normalised to a standard area unit. Where it is clear from the source, carbon is differentiated by the use of ‘OC’ for the organic form and ‘IC’ as the inorganic form. Total reported carbon includes both these forms. Sequestration rates (g OC m-2 yr-1), are sourced from the literature and annual sequestration capacity (t OC yr-1) is the product of rates and extents. For inorganic carbon (IC) calculations, carbon constitutes 12% of the molar mass of CaCO3 produced during calcification. Inorganic carbon values are presented as annual inorganic carbon production rates (g IC m-2 yr-1) and inorganic carbon production capacities (t IC yr-1) for the calcifying aggregations.
Table 1. Parameters and associated units adopted for this review.
Parameter | Unit | Abbreviation |
---|---|---|
Extent |
Hectare (100 ha = 1 km2) |
ha |
Carbon density |
Metric tonnes of carbon per unit area, for a given depth |
t C ha-1 |
Total standing stock |
Metric tonnes of carbon |
t C |
Sequestration rate |
Area-specific rate of annual carbon capture, generally as organic carbon |
g OC m-2 yr-1 |
Sequestration capacity |
Metric tonnes of carbon captured annually, generally as organic carbon |
t OC yr-1 |
Production rate |
Area-specific rate of annual carbon captured by kelp as organic matter |
g OC m-2 yr-1 |
Production capacity |
Metric tonnes of carbon captured annually by kelp as organic matter |
t OC yr-1 |
Inorganic carbon production rate |
Area-specific rate of annual calcium carbonate production * 12% |
g IC m-2 yr-1 |
Inorganic carbon production capacity |
Metric tonnes of calcium carbonate captured annually * 12% |
t IC yr-1 |
- |
Megatonnes (t x 106) |
Mt |
2. Vegetated Coastal Habitats
2.1 Machair
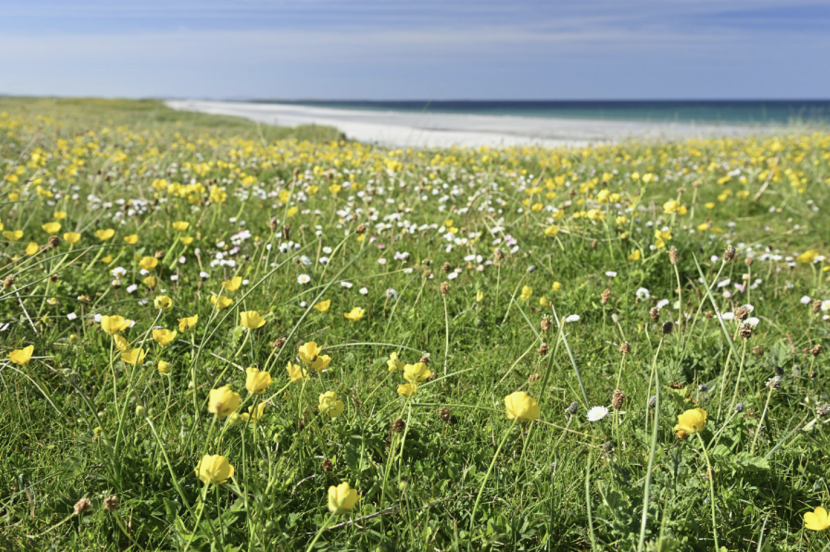
Machair is a distinct form of dune grassland found on shell-rich sands, unique to the north-western seaboards of Scotland and Ireland (Angus and Hansom, 2021). This restricted distribution results in machair having a considerably lower contribution to global blue carbon stores, when compared with other coastal habitats. Machair is a highly dynamic habitat, varying spatially and temporally in response to climate variations and changing land-use. Separating machair sites from hindshore can be difficult, especially as there is often a continuum between distinct areas of each (Dargie, 2000), which again poses issues when assessing habitat extent.
Distribution and extent of machair
Approximately 20,000 hectares (ha) of potential machair habitat has been estimated within Scotland, comprising 67% of the total global distribution (Jones et al., 2011; Dargie, 2000). A recent study estimates a more conservative figure for Scottish machair of 11,680 ha (Burden et al., 2020). Of the Scottish machair, the largest extent is found on South Uist, designated as a Special Area of Conservation (SAC) and spanning the whole length of the island, up to 2 km inland (Dargie, 2000).
Blue carbon contributions from machair
For the South Uist SAC, Burrows et al. (2017) determined a geological total carbon stock of 4,429 tonnes (t), within the top 0.1 m of sediment, split between 1,985 t OC and 2,444 t IC. Applying the area-specific estimate of carbon (19.5 t C ha-1, cited as 1,950 t km-2 in Burrows et al., 2017) to the conservative prediction of Scottish machair extent produces a total carbon stock estimate of approximately 228,000 t. This can be considered long-term storage within the sediment. This value was estimated through broad classifications of sediment type with carbon stock values based on limited Scottish data availability. As sediment from machair habitat was not sampled, this carbon stock estimate should be used with caution and is not used further in this review. The biological carbon standing stock of machair was not considered in this study.
Previous studies have considered the organic carbon stored in machair biomass and soil, with an estimated total figure of 683,000 t C sequestered in Scottish machair systems (Beaumont et al., 2014). This estimate uses the %loss on ignition (LOI) of eight soils sampled from six machair sites down to 0.15 m in the Outer Hebrides in 2010 (Beaumont et al., 2014). However, it assumes the same above and below-ground plant carbon content and biomass as those of fixed dune grassland due to a lack of habitat-specific data. The overall figure was based on a machair extent estimate of ~20,000 ha, which is likely an overestimate of current habitat. Applying the more recent extent estimate of 11,680 ha to values from Beaumont et al. (2014), provides a revised figure for total organic carbon stored in Scottish machair biomass and soil of 409,000 t OC. Dividing the total stock by total extent gives an average rudeimentary carbon density of 35.02 t OC ha-1. This value should be used with some caution given the assumption made that fixed dune grasslands and associated data are representative of machair habitat. There is also likely to be spatial heterogeneity in the organic carbon density values of machair systems due to variation in vegetation type and management that is not considered within an average value.
From this revised figure, the total organic carbon soil stock down to 0.15 m represents 224,000 t OC, using the value for soil carbon density of 0.0128 g C cm-3 from Beaumont et al. (2014). This excludes the carbon stored in machair biomass and is therefore agreeable with the estimate from Burrows et al. (2017), but it should be noted that carbon stock was measured down to different sediment depths.
No long-term carbon sequestration rates have been estimated for machair, so a mean rate (± s.d.) of 34.9 ± 15.7 g OC m-2 yr-1 was assumed, based on the ratio of soil carbon measurements and ecological similarities to sand dune grasslands (Beaumont et al., 2014). The values presented by Beaumont et al. (2014) likely hold large uncertainties due to the small sample size and assumptions adapted from sand dune habitats. Despite the limited sample size, the carbon stock estimate of 409,000 t OC within Scottish machair systems (Beaumont et al., 2014) is likely more reliable than the value derived from Burrows et al. (2017) data, due to the latter using broad classifications of sediment type instead of samples from machair habitat.
Threats to machair
Comparing data from historical (1880s–1970) to the recent period, erosion extent of machair habitats in the Western Isles has decreased from 16% to 13% and accretion has increased slightly from 11% to 12%. However, results differ between sites and the average rate of retreat has increased from 0.6 to 1.3 m yr-1 since the historic period, whilst accretion has decreased slightly from 0.9 to 0.8 m yr-1 (Burden et al., 2020). This potential imbalance may be further exacerbated by climate change and altering agriculture or land-use practices.
As machair habitats tend to be low-lying, they are particularly vulnerable to Relative Sea Level Rise (RSLR) (Angus and Hansom, 2004). Future losses of machair are broadly predicted to be 6% by 2060 as a result of RSLR (Beaumont et al., 2014; JNCC, 2007). Extreme storms, such as that of January 2005 in the Uists, also have the capacity to breach the dune ridge and flood the machair interior (Angus and Rennie, 2014). As storm frequency and severity may increase with the changing climate, this is likely to have an increasing impact in unison with RSLR (Burden et al., 2020). Actions that enhance the resilience of protective coastal habitats will also help safeguard the carbon stored in machair habitats (Angus, 2018).
Grassland improvement may also affect machair, especially in Tiree and parts of Orkney (Angus et al., 2011). Other pressures may stem from changing agriculture practices and additional grazing by introduced rabbits (Jones et al., 2011). Machair systems as a whole may yet prove resilient to climate change, as they have evolved over millennia in tandem with RSLR and human management (Angus, 2018; Angus and Hansom, 2021; Burden et al., 2020).
Future research on machair
Whilst evidence gaps and large uncertainties exist for machair – current extent, rate of habitat loss and sequestration rates – the relative contribution of these factors to Scottish blue carbon may not be significant compared to other coastal habitats. From a blue carbon perspective, more focus should perhaps be given to improve the understanding of other coastal habitats instead.
2.2 Sand dunes
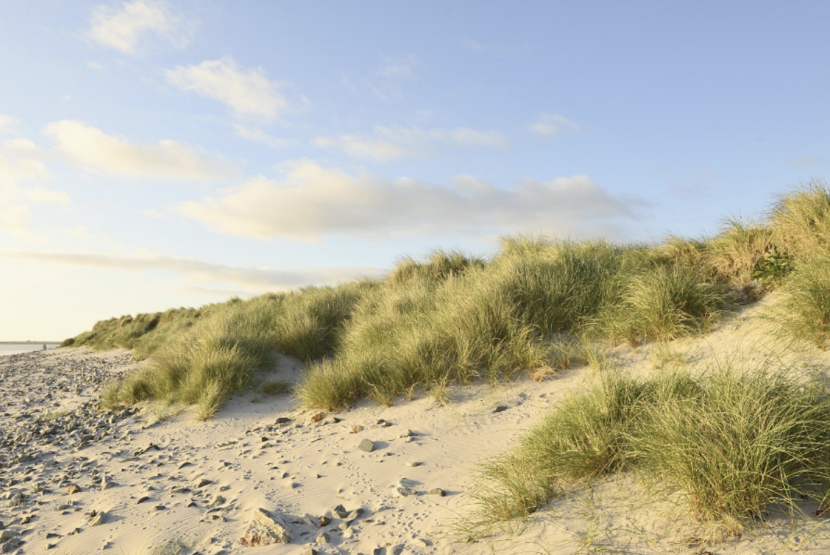
Coastal sand dunes are formed initially by sand blown inland off beaches that becomes trapped by vegetation, shell or clasts of rock. Embryo and mobile dunes start to form on the foreshore when the salt-tolerant pioneer species, such as marram grass, fix roots into the bare sand. As vegetation increases, so does sand capture, and these starting dunes grow becoming subsequently stabilised over time by vegetation, forming semi-fixed and eventually fixed dune. These habitats are dynamic systems that are constantly evolving, when free of human pressure.
Distribution and extent of sand dunes
Scotland has approximately 70% of the UK’s sand dunes by area (Angus et al., 2011). A national sand dune vegetation survey completed between 1994 and 1998 estimated there were 50,000 ha of “potentially vegetated sand”, which included machair and saltmarsh habitat (Dargie, 2000). The data collected from this survey have since been collated into the Habitat Map of Scotland (HabMoS) and during this process were reclassified into Annex I habitat types (Strachan, 2017). The resulting combined polygon extent for fixed (Annex I habitat: H2130, H2140, H2150), mobile (Annex I habitat: H2110, H2120), and dune slack habitat (Annex I habitat: H2190, H2170) cover a total area of 15,022 ha, which is the current best available Scottish sand dune extent. Values for extent of sand dune habitat are subject to high uncertainty due to the dynamic nature of these coastal systems. Continuing habitat losses are also associated with coastal development, plantations, invasive species and conversions to improved grassland (Angus et al., 2011). Data trends have shown a continuous decline in extent within Scotland since 1900 (Beaumont et al., 2014). The conservative value of 15,022 ha sand dune extent will be used to estimate total carbon stocks and densities.
Blue carbon contributions from sand dunes
The first organic carbon stock estimate calculated for Scotland’s sand dune systems used the full areal extent of ”potentially vegetated sand”, 50,000 ha, reported in the national Scottish sand dune vegetation survey (Dargie, 2000). The total estimated stock of 2,023,000 t OC that was reported in this study considered the OC component of above and below ground biomass, as well as that stored within the top 0.15 m of soil (Beaumont et al., 2014). Carbonates had been removed using acid prior to CSN analysis. However, this initial stock value is considered likely to be an over-estimate given the habitat reclassification process described above which has estimated a much lower extent value. The carbon data for above and below ground biomass used by Beaumont et al., (2014) were based upon samples from 11 dune systems around England and Wales (Jones et al., 2004), with soil samples obtained from three sites in the Outer Hebrides for comparison (Jones et al., 2004). As the only known OC data, this review has calculated a revised carbon stock value for an extent of 15,022 ha of sand dune area, which is further broken down into extents of fixed (11,938 ha), mobile (1,576 ha), and dune slack habitat (1,508 ha) as explained above. Using the relevant soil bulk densities values for each habitat (Beaumont et al., 2014), ranging from 7.17-63.7 t OC ha-1, a carbon stock of ~ 504,895 t OC is estimated for the top 0.15 m of soil. Additionally, taking the vegetation biomass and bulk % OC content values for the respective dune habitats (Beaumont et al., 2014), an estimated 78,581 t OC is stored within the above ground dune vegetation, and a further 169,478 t OC in the root biomass. The total carbon stock for Scottish sand dune systems thus calculated within this review is 752,954 t OC.
A chronosequence study to assess soil development over 140 years was conducted for Newborough Warren, a large dune system in North Wales (Jones et al., 2008). It found mean carbon sequestration rates (± s.d.) of 58.2 ± 26.2 g OC m-2 yr-1 in dry dunes and 73.0 ± 22.1 g OC m-2 yr-1 in wet dune slack habitats. Using the HabMoS database figures, the dry dune vegetation (assumed to comprise of mobile and fixed dune) makes up approximately 90% of Scotland’s sand dune vegetation, with approximately 10% of dune slack. Taking these data, a proportional average sequestration rate for sand dunes in Scotland equates to approximately 60 g OC m-2 yr-1. This does not take into account wetland habitats such as wet grasslands, mire, swamp and wet heath. Using the weighted average sequestration rate, an annual sequestration capacity of ~ 9,013 t OC yr-1 is estimated for Scotland’s sand dune habitat.
Applying sequestration values derived from these data to Scottish dune systems results in large uncertainties, as sand dunes are highly diverse systems. Heterogeneity arises from slope gradients, the degree of grazing or disturbance by animals, groundwater chemistry and differences in successional age (Everard et al., 2010). Another important factor is pH, which is influence by mineralogy. The mineralogy of Scottish sites varies greatly, with some dune systems comprised of fully calcareous shell-deposits (Everard et al., 2010). Unless shell content is renewed, leaching will reduce the calcareous content over time, with this applying to machair systems also. This likely increases uncertainty when using data from other areas in the UK. Thus, a great deal of caution should be taken when applying these estimates, for both carbon storage and sequestration to Scottish sand dunes.
Threats to sand dunes
As noted previously, areal extent of dunes has been lost historically to coastal development, forestry plantations, and conversions to improved grassland (Angus et al., 2011). Coastal erosion coupled with RSLR may also affect dune extent and sequestration potential, with an expected 6% loss of habitat across the UK by 2060 (Beaumont et al., 2014; JNCC, 2007). However, the proportion of habitat lost is predicted to be greater for dune systems in England compared to other regions (Beaumont et al., 2014). Regardless of whether global net zero CO2 emissions by 2100 are achieved, RSLR is predicted to continue for centuries (Wong et al., 2014), however its impacts on sand dunes are unpredictable. Erosion is a natural part of dune dynamism, which results in a redistribution of sand elsewhere along the coast. However, the physical removal of sand from the system without replacement will reduce the system’s resilience. A focus on reducing manageable human pressures, such as through control of invasive species, will help to build resilience on a local scale by enabling native biodiversity to flourish (Angus and Hansom, 2021).
Other climate-driven pressures could threaten sand dunes and their associated blue carbon capacity. Between the years 1990 and 2012, there was a 30% loss in dune slack extent at certain protected sites in England, with evidence showing that dune slacks are drying out (Burden et al., 2020). This will affect the plant community composition and biodiversity supported. Plant species trap sand in different ways, leading to different dune formations (Ruggiero et al., 2018), which may influence overall dune stability. Rainfall patterns are likely to be affected through climate change, leading to soil moisture deficits and summer droughts (Burden et al., 2020). The proportion of mosses and lichens have been shown to increase under predicted dryer conditions (Bartholomeus et al., 2011), as bare soil and non-rooting species have a much lower evapotranspiration compared to vascular plants. This shift to non-rooting species may impact dune stability and surficial carbon stocks.
One climate change sensitivity study used a bioclimatic model linking the current distribution of 84 plant species, including coastal vegetation, that characterise Danish and European habitats to climate (Normand et al., 2007). Predictions were then made about where climatically suitable habitat would exist in 2100 (Normand et al., 2007). Under a business as usual climatic scenario, 75-85% of species showed a decline in Denmark (Normand et al., 2007), which is at a similar latitude to northern England and Scotland. However, a study that revisited 89 coastal sites in Scotland after 34 years, found very little evidence of shifting species compositions (Pakeman et al., 2015). The authors postulated that no apparent shift in dune vegetation between 1976 and 2010 could be due to a number of reasons including, a general resistance of dune vegetation to change, management practices that have prevented the dominance of species migrating from the south, or dispersal limitations of vegetation in often fragmented coastal sites. The discrepancy in the timings of these two studies raises questions about whether the effects of climate change on coastal dune vegetation remain to be seen and whether they will become more apparent as change continues.
Grazing can also influence dune system functioning. A higher diversity of plant species was associated with a higher density of rabbits at a National Nature Reserve in north-east Scotland (Patterson, 2019). This was highlighted as a positive pressure in this case, with the presence of rabbits encouraging the growth of rich, diverse moss, preventing coarse grasses and gorse shrub from becoming dominant and crowding out sensitive plant species (Patterson, 2019). Similarly, a study at Newborough Warren, North Wales, found an increase in plant species richness in dry dunes under a grazing regime by large animals (Plassmann et al., 2010). This was deemed a positive pressure, given the enhanced abundance of positive indicator species for habitat condition. No change in vegetation community type in dune slacks was recorded (Plassmann et al., 2010). Grazing was shown to have no significant effect on soil organic matter content, bulk density or total carbon stock at the same site (Ford et al., 2012). As Scottish sites vary greatly, caution should be placed when applying results from other regions. To the best of our knowledge, no studies have assessed how grazing impacts the blue carbon service of dune systems in Scotland, and represents a gap in current knowledge.
Historically, conifer plantations were planted across UK dune systems in an attempt to prevent erosion. Whilst the trees stabilised the dune systems, the natural process of sand replenishment was interrupted, causing a loss of dynamism and protective functioning of the dunes (SNH, 2017). Additionally, the net effect of dune forestry on wild species diversity is negative, with invertebrate diversity lower in the afforested areas of the dune system at Newborough Warren (Loxton, 2014). Restoration to near-natural sand dune habitats has gained interest as a result (SNH, 2017). Forestry on dunes increases the carbon stocks in soils and above-ground (Hill and Wallace, 1989), whilst also adding to the amenity value by providing diverse forest-related activities (Jones et al., 2011). However, in the interests of the natural functioning of dunes, the presence of non-native tree plantations should not be considered as a climate solution, because of the negative impacts on dune biodiversity and dynamism, which is essential for adapting to climate change (UKCEH, 2021).
Future research on sand dunes
Habitat extent data collected during the Scottish Sand Dune Vegetation Survey (Dargie, 2000) is unlikely to represent the current spatial extent and location of dunes due to the dynamism of these habitats. An initial scoping study could look at a representative set of dune systems around the Scottish coastline to evaluate the level of change since the survey to determine the need for and / or inform the requirements of any future national survey effort. This would act as a baseline for habitat change and help direct management. Sand dunes represent a large proportion of coastal area compared to other Scottish blue carbon habitats, thus prioritisation of future research in these systems should be considered.
From a blue carbon perspective, Scottish focused sampling should be conducted to estimate more accurately the total carbon storage and sequestration capacity of Scotland’s dune systems. There is a research need to better understand the pH and mineralogy of soils, and how these factors may influence the blue carbon service, as similar factors have been found to influence blue carbon stores within saltmarshes (Miller et al., 2023). As climatic pressures increase, impacts on Scottish dune vegetation should be investigated to understand likely changes, which could also influence blue carbon sequestration potential. For instance, in the future, there are likely to be issues around water balance, with eastern areas predicted to become hotter and receive less rainfall in summer (Adaptation Scotland, 2022). The impact this has on dune slacks and the resulting blue carbon service should be investigated further.
Given the lack of Scottish specific research on how grazing regimes may influence the blue carbon service, this is another prominent evidence gap, which has implications for grazing management. The increase in frequency of severe weather events pose an increased risk to Scottish forests, particularly to conifer plantations along the coast, which have shallow root systems (Scottish Forestry, 2022). At coastal plantation sites, restoration back to sand dunes presents a growing opportunity for climate adaptation and resilience to the pressures of relative sea level rise. Further work should assess the impact on ecosystem services, including carbon sequestration, of restoring sand dunes from conifer plantations.
In the meantime, management should be focused on pressures that can be addressed by local policies and actions, as outlined in Angus and Hansom (2021). Interventions to increase the height and width of a dune ridge may be nature-based or manual. For instance leaving drift seaweed on the foreshore enables a natural trapping and accumulation of sand; alternatively, manual sand recharges using machinery can reduce vulnerability to erosion, however this may be short-term due to ongoing sediment movement. Implementing additional drain outflows higher in the tidal frame can help to manage hydrology. These interventions should be focused in areas where asset protection from coastal retreat is considered high priority. Management of visitors to popular coastal dune systems can also be considered; restricted access tracks through the dunes for visitors, for instance at West Sands in St Andrews has shown considerable success in reducing the impact of fragmentation caused by ad hoc paths on the dune habitat condition (JNCC, 2021). The Dynamic Coast project has produced an evidence-based resource that highlight areas of the Scottish coastline most vulnerable to erosion to support decision-makers (Dynamic Coast, 2023).
2.3 Saltmarsh
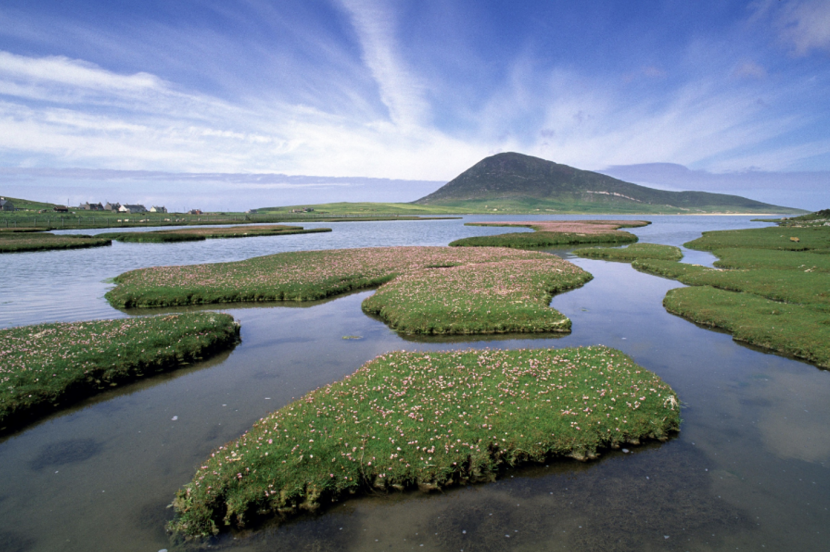
Saltmarshes are dynamic, soft-sediment landforms found along the coastline, generally in sheltered bays. Saltmarsh habitats are situated within and above the intertidal zone and are driven by saline conditions. They are characterised by a cover of halophytic plants that evolve from the pioneer zone, which is inundated daily by the tide, to the upper marsh zone that may only be flooded by saline water at the highest astronomical tides (Ladd, 2021). Saltmarshes develop from adjacent unvegetated tidal flats and can undergo natural cyclic periods of expansion and contraction over time scales of decades to centuries (Fagherazzi et al., 2020). In the UK, long-term marsh expansion and contraction has been linked to sediment supply (Ladd et al., 2019) and/or rates of RSLR (Horton et al., 2018).
Distribution and extent of saltmarsh
During 2010 - 2012, a national Scottish saltmarsh survey was undertaken to map all saltmarsh habitat >3 ha in extent, which resulted in a total extent of 5,840 ha recorded (Haynes, 2016). The west coast of Scotland is dominated by smaller loch head and perched saltmarshes, while the east coast is characterised by embayment, fringing, and back barrier marshes. It should be noted that although widely reported as the current extent of saltmarsh within Scotland (Austin et al., 2021; Miller et al., 2023), this figure is likely to be an underestimate noting that fringe saltmarshes (<3 ha) were not included (Haynes, 2016). Saltmarsh is a dynamic habitat, particularly in the pioneer zone, and can undergo lateral changes with adjacent mud or sandflats (Ladd et al., 2019). The main concentration of saltmarshes in Scotland cover the low-lying firths of the eastern and south-west region (Haynes, 2016). Scottish saltmarsh accounts for approximately 13% of the UK total extent (Smeaton et al., 2022).
Blue carbon contributions from saltmarsh
Saltmarshes are one of the most effective blue carbon habitats, due to a combination of rapid carbon sequestration and storage capacity. Hypoxic conditions resulting from the inundation of seawater serve to reduce the decomposition rates of organic matter, increasing burial potential (Mcleod et al., 2011). Greenhouse gas fluxes from saltmarshes also vary as a function of salinity and sulphate concentrations within seawater, with higher salinity environments (>18 psu) likely to suppress methane emissions and support a net carbon sink status for the habitat (Kroeger et al., 2017).
In recognition of the important role that saltmarshes play in carbon cycling and storage, a number of recent studies have attempted to estimate the total carbon stocks at a regional and national scale within Scotland (Burrows et al., 2014; Beaumont et al., 2014; Porter et al., 2020; Austin et al., 2021). Generally, carbon stocks within saltmarsh are calculated for a standardised depth in surficial soils (10 cm depth), which allows for comparison with other blue carbon audits (Legge et al., 2020, Smeaton et al., 2022). The most recent carbon stock estimate for surficial saltmarsh soils in Scotland reported a value of 0.368 ± 0.091 Mt OC (10 cm soil depth), representing 16% of the saltmarsh soil carbon within Great Britain (GB) (Smeaton et al., 2022). This study used a combination of citizen science data and additional secondary data collected across the spatial range of GB’s saltmarshes to generate this estimate.
Although organic carbon is stored throughout all components of saltmarsh habitat, few studies have considered calculating full stock estimates including vegetation biomass and root material. One such study conducted for the UK in 2014, estimated a total Scottish saltmarsh habitat stock of 569,700 t C (0.57 Mt C) (Beaumont et al., 2014), however this value was extrapolated based on a limited number of data points collected from Welsh and English saltmarsh systems and for a total soil depth of 0.5 m. This full stock value has been revised recently using a Scottish-specific saltmarsh dataset, including measurements for OC in the vegetation, roots and total depth of saltmarsh soils, taken from four representative saltmarsh systems (Miller et al., 2023). Using a Bayesian statistical model, the measured stocks were scaled up for Scotland’s 240 mapped saltmarshes. Total carbon stocks were estimated as follows: 0.013 ± 0.0027 Mt C in the above ground biomass (vegetation), 0.088 ± 0.034 Mt C in the below ground biomass (roots), and 1.05 ± 0.21 Mt OC within the full saltmarsh soil profile, giving a fully inclusive stock of 1.15 ± 0.25 Mt OC. Soils therefore hold > 90% of the carbon stock within saltmarsh habitat. Variations in carbon stocks were seen regionally and between zones within the same saltmarsh system. Carbon was observed to vary across the saltmarshes as a function of biomass density, soil composition (sandy soils hold less carbon than muddy soils), and saltmarsh zone (high marsh zones hold more carbon in biomass and soils than low-mid marsh zones) (Miller et al., 2023), highlighting the importance of representative data for national-scale audits.
The average soil carbon density calculated for saltmarsh varies between studies and the depth profiled. A previous Scottish study reported soil densities of 36.4 - 65.7 t C ha-1 down to a depth of 10 cm and 54.6 - 98.6 t C ha-1 down to a depth of 15 cm (Austin et al., 2021). Smeaton et al., (2022) estimates an average C density for the top 10 cm of 6.31 kg C m-2, equivalent to 63.1 t C ha-1. However, in the most recent study by Miller et al. (2023), an average carbon density accounting for the full saltmarsh soil profiled was estimated to be 18.6 ± 3.9 kg C m-2, equivalent to 186 t C ha-1. This is substantially higher than the surficial estimates measured and is due to compaction of soils with depth leading to higher carbon densities that are not representative of 10 cm surface stocks (Miller et al., 2023). Further, soil types and saltmarsh location, which influences sediment accumulation rates, can also influence carbon density values (Smeaton et al., 2022). It is therefore essential to consider the depth range of any soil carbon stocks being reported to ensure comparable estimates are being made.
It has been suggested that saltmarshes are one of the most efficient habitats at storing carbon, with an average global sequestration rate of 210 ± 20 g C m-2 y-1 (Chmura et al., 2003). North American sites dominated this average and high variability was recorded, even within regions. This was likely due to the variation in soil compositions and tidal regimes, with interregional differences arising from combining values from peat-dominated freshwater ecosystems and increasingly minerogenic tidal marshes. Caution should be taken when applying this value to Scottish systems, as the largest Scottish marshes are estuarine in nature (Haynes, 2016), making up ~42% of habitat extent, followed by ~30% which are characterised as embayment saltmarsh (Austin et al., 2021). Generally, American marshes along the Atlantic coast tend to be organogenic, whilst European sites are predominantly minerogenic. This holds true for most Scottish saltmarshes, other than those in the Inner Forth and the Outer Hebrides, which tend to be peat-based and therefore more organic in nature (Angus, 2001; Smout and Stewart, 2012).
Sequestration rate estimates in UK saltmarsh have ranged from 64 to 219 g C m-2 yr-1, with typical values around 118.5 to 150 g C m-2 yr-1 (Cannell et al., 1999; Chmura et al., 2003; Adams et al., 2012; Beaumont et al., 2014), but these figures are largely based on English marshes. A recent study based on a Scottish specific dataset used a number of chronological markers to calculate sequestration rates, (described as organic carbon accumulation rates (OCAR)), for a representative suite of saltmarshes (Miller et al., 2023). Variable rates were observed between the saltmarshes and within the saltmarsh zones. The average high marsh sequestration rate of 71.5 ± 9.3 g C m-2 yr-1 is lower than the average low marsh sequestration rate of 103.4 ± 18.4 g C m-2 yr-1 due to less frequent tidal inundation (Miller et al., 2023). The estimated average sequestration rate across location and zones is approximately 87.45 g C m-2 yr-1. This is lower than the global average and regional average for the NW Atlantic and is attributed to the inclusion of the sand-rich marshes that are commonplace in Scotland (Haynes, 2016), which have shown to accumulate carbon at a slower rate than muddier systems (Miller et al., 2023). The authors estimate that an additional 4,385 ± 481 tonnes of OC are added to Scotland’s saltmarshes annually. The majority of this carbon, ~85%, originates from terrestrial or in situ sources (Miller et al., 2023). It was not possible to distinguish the source of the organic carbon any further using stable isotopes, and is a remaining gap in knowledge.
Threats to saltmarsh
The current extent of saltmarsh is much less than historical values. Prior to the 1980s, major losses of saltmarsh through land claim were widespread throughout the UK, utilising the land for agriculture, urban development and industrial purposes instead (Morris et al., 2004). Over the last 400 years, the Forth Estuary has lost 50% of its intertidal area to these activities (Hansom et al., 2001). Saltmarshes in the Clyde, Tay and Moray Firths have also experienced area loss (Hansom and McGlashan, 2004).
Habitat disturbance may lead to declines in the blue carbon service provided by saltmarshes. A study in St Joseph Bay, Florida, assessed 296 patches of saltmarsh vegetation loss caused by seagrass wrack accumulation (Macreadie et al., 2013). Levels of OC within the first 0.05 m of sediment were found to be approximately 30% lower from disturbed patches, compared to the surrounding undisturbed areas. This decline in the subsurface OC was due to a loss in the below-ground plant biomass, which would otherwise sequester carbon over the long-term. Whilst loss from seagrass wrack accumulation is not a prevalent threat to Scottish saltmarshes, vegetation loss can still occur from damage by grazing livestock (Burrows et al., 2014). This results in an increased algal coverage over disturbed patches. As algae do not have a root system, accretion rates are reduced. Thus, this observed decline in surficial OC may also occur in Scotland saltmarshes subject to overgrazing. Out of 249 Scottish saltmarshes surveyed during the national saltmarsh survey, 39 were reported as being overgrazed with 166 failing targets to achieve Favourable Condition under the Habitats Directive (Haynes, 2016). Additionally, there were 123 non-designated sites where overgrazing was considered to be the most significant pressure (Haynes, 2016).
The relationship between saltmarsh carbon sequestration and grazing has previously been studied, with contradictory results likely arising from geographic differences, importance of other abiotic factors, and differing grazing intensities or type of livestock (Muenzel and Martino, 2018). Grazed saltmarsh in Canada (Yu and Chmura, 2009) and the Netherlands (Elschot et al., 2015) had higher soil carbon contents compared to non-grazed sites, whilst those in Denmark had lower soil carbon content (Morris and Jensen, 1998). Non-grazed sites in Denmark were shown to have a 25% increase in sediment carbon storage compared to grazed areas (Morris and Jensen, 1998). A large-scale study in England and Wales found grazing to be an insignificant impact on soil carbon stocks or overall carbon sequestration rates across 22 saltmarshes, with abiotic factors deemed largely responsible for below-ground processes instead (Kingham, 2013; Harvey et al., 2019). A meta-analysis determined that grazing was likely to reduce soil carbon in American saltmarshes, with no consistent effect in European studies (Davidson et al., 2017), potentially due to American marshes being predominantly organogenic compared to the generally minerogenic European sites.
In addition to impacting the carbon sequestration service, overgrazing also influences the biodiversity of saltmarshes, with biodiversity generally higher under a light grazing regime, which replicates a natural system (Adnitt et al., 2007). Trampling by large grazers is another related pressure, with a study in the Netherlands reporting increased bulk density of the sediment in the presence of large grazers (Elschot et al., 2013). This in turn reduces marsh accretion rates, which could have implications for how saltmarshes keep pace with sea level rise (Elschot et al., 2013).
Other anthropogenic pressures threatening marshes include pollution, high nutrient loads from agricultural activities, and waste disposal (Burrows et al., 2014).
Restoration of saltmarsh
Managed realignment of saltmarshes has been a popular approach in England to restore and create saltmarsh habitat. To understand the impact of managed realignment on biogeochemical cycling and carbon sequestration, a study compared natural saltmarshes with restored agricultural areas 15 years after realignment in Tollesbury, Essex (Burden et al., 2013). It found that whilst a reversion to the natural biogeochemical functioning is possible, it is likely to be a slow process with an estimate of 100 years for the restored saltmarsh to accumulate the same amount of carbon stored within the natural site. This equates to a sequestration rate of 92 g C m-2 yr-1 (Burden et al., 2013), which is considerably lower than the average UK sequestration rate quoted earlier (118.5 to 150 g C m-2 yr-1).
A holistic approach looking beyond blue carbon should be considered in management decisions, as restoration would have wider benefits for biodiversity and people. Managed realignment of saltmarshes may increase the following ecosystem services: climate change mitigation, nature-based recreation and flood risk protection. See Macdonald et al. (2017) for an overview of the potential wider ecosystem service benefits associated with proposed managed realignment schemes in the Inner Firth of Forth.
In 2003, Scotland’s first coastal realignment project was undertaken in Nigg Bay, Cromarty Firth (Elliot, 2015). Other Scottish managed realignment projects have been carried out including two on the Firth of Forth – Skinflats (11 ha) and Kennet Pans (8.2 ha) – as well as a small project (0.3 ha) in Montrose Basin. Project details are recorded on the Online Marine Registry (OMReg), a database showcasing completed coastal habitat creation schemes within the UK. A review of these completed projects could be used to evaluate the success of managed realignment in Scotland from a carbon perspective. A feasibility study has been carried out within the Firth of Clyde to identify further potential managed realignment sites there (Hansom et al., 2017). This study concluded that infrastructure placed limits on the implementation of a managed realignment scheme along the Inner Clyde North, but a number of other sites showed realistic opportunities to increase current saltmarsh extent.
To date, only small-scale projects have been carried out within Scotland. A recent national review assessing the extent of opportunities for managed realignment to create suitable areas for saltmarsh habitat identified 15 potential Scottish sites (Austin et al., 2022). The study concluded that there is significant potential within Scotland for managed realignment that would contribute to blue carbon gains. However, significant knowledge gaps were identified including a lack of evidence for carbon accumulation rates and methane fluxes within restored sites, and a lack of understanding of the natural functioning of saltmarsh ecosystems, particularly relating to their likely geomorphic response to future sea-level change. Nevertheless, this work has provided a baseline of opportunities nationally for managed realignment projects. Similar work has been conducted in England and Wales, identifying suitable sites and developing data layers to aid searches for potential sites for restoration and creation (MMO, 2019).
Transplantation of saltmarsh vegetation is another method of restoration. Initial small-scale trials have taken place in the Eden Estuary on the east coast of Scotland to assess the functionality of restored saltmarsh and develop best practice restoration methodologies (Maynard et al., 2011; Taylor et al., 2019; Maynard, 2020). Taylor et al. (2019) found that vegetative transplants can enhance sedimentation in eroded fringe saltmarsh, with four-year-old transplants of Bolboschoenus maritimus having similar sedimentation rates compared to natural sites. However, transplants may not be as effective as retaining the sediment compared to natural sites, at least within the formative years of being planted. Transplantation of saltmarsh is still a growing area of research (e.g., Maynard, 2020), but has potential implications for saltmarsh resilience to relative sea level rise and an increase in storminess, with the latter affecting timing, quantity and potentially, sources of sediment (Burden et al., 2020).
Future research on saltmarsh
Recent research has improved the evidence base of carbon sequestration and storage in contrasting Scottish saltmarshes as a function of the soil type, physical setting, habitat component (biomass, roots, spoils), vegetation type, and marsh zone (Miller et al., 2023). The sources of organic carbon and burial rates were also quantified at these contrasting sites. This study has provided a better understanding of the factors influencing deposition, accumulation and burial of organic carbon within Scottish saltmarshes. Evidence gaps still exist for greenhouse fluxes within saltmarshes, the fate of carbon as it is transported within the system, and sediment transport dynamics (Austin et al., 2022). Additionally, the blue carbon service provided by mudflats should be investigated, as the extent of saltmarsh to mudflat varies in an alternative stable state.
As anthropogenic and climate pressures are likely to increase in the future, saltmarshes may shift from long-term carbon stores to sources of carbon emissions. Managed realignment projects may therefore be a viable proposition to partially offset this potential carbon storage loss. Further work is needed to assess the capacity for restoration in Scotland, given constraints posed by the proximity of railways and other built infrastructure to saltmarshes. Research is also needed to better understand the blue carbon service of restored sites, especially those in Scotland.
The perturbation of existing stores is another knowledge gap that should be targeted, with management aiming to reduce the pressures to saltmarsh ecosystems. Given the occurrence of overgrazed saltmarsh on the west coast and lack of Scottish specific research, assessments into the impacts of different grazing regimes and intensities on the Scottish saltmarsh blue carbon store should be further investigated.
3. Nearshore Habitats
3.1 Seagrass beds
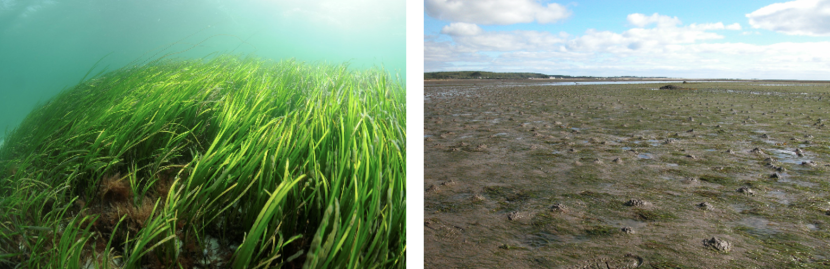
Seagrasses (also known as eelgrass) are marine flowering plants that can form extensive beds or meadows in shallow waters, with those in Scotland typically occurring from the lower saltmarsh limit into the sublittoral zone (Burrows et al., 2014). The seagrass species in Scotland are Zostera marina, Zostera noltii and Ruppia maritima.
Distribution and extent of seagrass beds
Seagrass beds are particularly well developed in Scotland, with subtidal beds predominantly occurring along the west coast and intertidal beds on the east coast. There are no complete estimates of seagrass coverage within Scottish waters. However, there have been efforts to develop polygons from point data for seagrass beds. Using data from the Geodatabase of Marine Features in Scotland (GeMS), 1,088 ha of Z. marina and 182 ha of Z. noltii have been estimated (GeMS_V8_i24 – accessed Jan 2021). This does not include the Cromarty Firth, which is known to have vast beds of Z. noltii and patches of intertidal Z. marina (RSPB, 1995). During 2015, SEPA assessed this area, specifically Udale Bay and Nigg Bay, for seagrass. The survey was conducted during the summer months at peak seagrass growth, using GPS data to construct spatial maps. The area covered was limited between mean high water and mean low water on a spring tide. Approximately 324 ha were mapped across both bays (Marine Scotland, 2020). Historically, the Cromarty Firth has been estimated to have 1,200 ha of seagrass extent (RSPB, 1995). There is a temporal aspect to be considered with these records, particularly as this habitat can be ephemeral and it is not known whether the beds remain without revisiting them. Thus, the RSPB (1995) Cromarty Firth estimate is likely less reliable than more recent surveys and will not be used in this review. Combining the data from GeMS and the surveys from SEPA, represents an estimate of 1,504 ha of Scottish seagrass beds. This likely represents an underestimate, as the records in GeMS are subject to limited survey effort. There are potentially undiscovered Scottish seagrass beds and current point locations significantly underestimate the likely presence of larger seagrass beds around the point.
Burrows et al. (2014) estimated a total extent of 1,590 ha in Scotland, which incorporated the RSPB (1995) Cromarty Firth estimate, along with extrapolating known records that lie within existing mapped areas. At that time, only 10% of Scottish seagrass beds were within mapped areas. Given the mapping efforts since then, the Burrows et al. (2014) estimate is likely less reliable than the value derived in this review of 1,504 ha. However, it is acknowledged that this is likely to be an underestimate.
Blue carbon contribution of seagrass beds
Research on seagrass bed ecosystem function, processes and services largely comes from studies conducted outside of Scottish waters. Previous studies have predominantly focused on two main species – Posidonia oceanica and Cymodocea nodosa – with a focus on Mediterranean and Australian sites (Fourqurean et al., 2012). The global average for the stock in the sediment (top 1 m) of seagrass habitats is 165.6 t C ha-1 (Fourqurean et al., 2012). However, this estimate incorporates many seagrass species, including Posidonia, which have a robust, slow growing root structure compared to Zostera species, which are relatively fast growing. Applying this global average may not be representative of Zostera meadows. However, Röhr et al. (2018) collected samples from 54 Z. marina beds across eight ocean margins and seas during the summer months. Projected OC stocks over the top 1 m of sediment ranged between 23.1 and 352 t C ha-1, which is within the range of other seagrass species estimates and other blue carbon ecosystems (Röhr et al., 2018). However, the high variance does highlight the need for Scottish specific estimates, rather than applying regional or global averages.
A more focused study utilising data taken during the summer from 13 UK sites of Z. marina found a mean sedimentary stock of 140 ± 73.32 t C ha-1 over the top 1 m, extrapolated from cores of 0.3 m depth(Green et al., 2018). This is within the range derived from Röhr et al. (2018), which is reassuring. Additionally, OC in intertidal seagrass sediments (top 0.5 m) was estimated for both Z. marina and Z. noltii beds across ten Scottish estuaries. Large spatial variation in OC was recorded within the estuaries which ranged from a minimum of 14.94 t C ha−1 at the Moray Firth to a maximum of 105.72 t C ha−1 at the Firth of Forth, giving a mean value (±SD) of 54.79 ± 35.02 t C ha−1 across the 10 estuaries sampled (Potouroglou et al., 2021). The study collected 92 cores and each sample was stratified by core depth, with a higher density of sampling in the shallower half of the core. Utilising sample sizes above 40 cores and incorporating a stratified approach, has been shown to reduce variability significantly (Young et al., 2018). Extrapolating this figure to a 1 m depth through simple multiplication provides a value of 110 t C ha-1 (Potouroglou et al., 2021); however, this estimate assumes consistent sediment type and associated OC values from 0.5 m – 1 m depth. Extrapolation through this method has been shown to have a strong correlation to actual carbon content values, although applying a log-linear approach to the carbon content may hold slightly more confidence (Young et al., 2018). This latter figure is also agreeable with the values derived from Röhr et al. (2018), indicating that it is a relatively robust estimate.
Utilising the mean value from Potouroglou et al. (2021) of 54.79 ± 35.02 t C ha−1,and extent estimate from this review, 1,504 ha, provides a total stock of sedimentary carbon in Scottish seagrass meadows down to 1 m of approximately 0.17 Mt (165,440 t C). Uncertainty in the extent estimate may lower the confidence in this value. Variation of carbon storage between sites will also impact the confidence of this estimate.
For carbon capture of seagrasses, an average net sequestration rate is estimated at 83 g C m-2 yr-1 (Duarte et al., 2005). A review by McLeod et al. (2011) calculated a higher mean sequestration rate of 138 g C m-2 yr-1. Both values are global estimates, incorporating a wide range of seagrass species, including tropical, which are highly productive. The increase is from incorporating a higher estimate (160-186 g C m−2 yr−1), combining seagrass net community production and the carbon derived from other sources, which is subsequently trapped in their sediments (Duarte et al., 2010; Kennedy et al., 2010). Organic material that is not buried in situ is likely exported to adjacent habitats, including seagrass meadows, where it is subsequently buried (Kennedy et al., 2010). Thus, this may be considered a more robust estimate for seagrass contribution to blue carbon.
However, these global values are likely to be overestimates for Scottish seagrass meadows. Whilst no studies have been conducted within Scotland, sequestration rates have been calculated for six seagrass sites within the Solent region in England (Lima, 2020). Study sites encompassed seagrass habitats from both muddy and sandy substrates, including Zostera spp. and Ruppia spp. meadows. The average sequestration rate was calculated as 67.91 ± 32.39 g C m-2 yr-1 across the six sites. Combining this value with the previous estimate for habitat extent, Scottish seagrass meadows have an approximate total carbon sequestration capacity of 1,021 t C yr-1.
Threats to seagrass beds
Seagrass beds are considered marine conservation priority habitats; as an OSPAR threatened and/or declining habitat, Priority Marine Feature (PMF) in Scotland, and as a protected feature within Marine Protected Areas (MPAs). As a PMF, seagrass beds also receive a degree of protection outside of MPAs through General Policy 9 of Scotland’s National Marine Plan, which states that – Development and use of the marine environment must not result in significant impact on the national status of Priority Marine Features.
Seagrasses are in decline globally, with a loss in extent of 7% yr-1 since 1990 (Waycott et al., 2009). This figure is based mainly on sites within Europe, North America and Australia. Historically, seagrass meadows in the UK suffered a major die-back due to a wasting disease, with at least 44% of the UK’s seagrasses lost since 1936 (Green et al., 2021). The decline of Z. marina in the UK has been estimated in the range of 25-49% between 1980 and the early 2000s (Hiscock et al., 2005). A key threat to seagrass in the UK is encroachment by the non-indigenous invasive cord grass, Spartina spp. (d’Avack et al., 2014). Seagrass beds are sensitive habitats, particularly to smothering, nutrient enrichment, physical disturbance and changes in water flow (d’Avack et al., 2014). Anthropogenic activities such as industrial runoff, development and dredging threaten seagrass meadows as a result of this sensitivity (Grech et al., 2011). Disturbance to the bed can cause fragmentation and mobilisation of sediment, which can subsequently prolong recovery times. Fragmentation may be considered to be more damaging than the total area of seagrass loss (Kent et al., 2021).
As storm frequency and severity are expected to increase with climate change, this may impact seagrass through increased turbidity and decreased water quality. In the case of pulsed turbidity events following the passage of a tropical storm in Australia, 100,000 ha of seagrass was lost (Preen and Marsh, 1995). The rise in seawater temperature associated with climate change may also pose threats, as P. oceanica suffered increased mortality rates resulting from a 3°C increase (Marbà and Duarte, 2010). There is also a potential positive impact associated with rising temperatures as it may increase seed germination and senescence in the winter may be reduced (Hootsmans et al., 1987). Furthermore, rising sea levels may impact seagrass through increasing water depth and reducing the light availability at the sea bed. Since seagrasses have high light requirements (Orth et al., 2006), this could hamper seagrass growth, production and potential carbon sequestration. However, rising sea levels also have the potential to shift beds inland (MCCIP, 2018). Ocean acidification may also have a beneficial impact to seagrasses as survival, photosynthesis, growth and proliferation have been shown to increase at warm temperatures under certain acidification scenarios (Repolho et al., 2017; Zimmerman et al., 2017).
Seagrass bed restoration
Global declines in seagrass beds along with a growing appreciation for the value of such habitats has stimulated interest in seagrass restoration in a number of countries and regions. However, a study by van Katwijk et al. (2015) found a success rate of only 37% for seagrass restoration projects globally. One of the main factors that cause a restoration effort to fail is due to unsuitable environmental conditions, with reduced water quality leading to poorer success rates (van Katwijk et al., 2015). During the site-selection process, proposed sites should be studied in detail to determine the cause of any declines in bed condition or extent. It is important that any existing pressures are first removed, as the restoration effort is likely to fail otherwise. Through a range of selective measures, research (e.g. on seed viability and habitat suitability) and guidelines, seagrass restoration projects may be successful. Restoration efforts are more likely to be successful as techniques develop and improve, however the method deployed will vary on a site-basis basis. One novel method using ‘Bags of Seagrass Seeds Line’ (BoSSLine) developed by Swansea University and Project Seagrass, plants seeds within hessian bags. This method has been shown to reduce seed burial and consumption by predators in the early stages of planting, increasing chances of success (Unsworth et al., 2019). Other methods include “Dispenser Injection Seeding” (Govers et al., 2022) and the use aquaculture nurseries to germinate plants before manual transplantation (Chambers et al., 2022b).
Restoration of subtidal seagrass beds has a range of potential benefits, from providing enhanced fish nursery grounds to offering blue carbon services (Duarte et al., 2020; Greiner et al., 2013). Restored Z. marina beds have carbon sequestration rates of 36.68 g C m-2 yr-1 10 years after seeding in the Virginia coastal bays (Greiner et al., 2013). After 12 years, the beds were predicted to accumulate carbon at a rate that is comparable to natural seagrass beds. This shows that restoration has the potential to enhance blue carbon stocks, although further research into Scottish specific dynamics is required.
Two main techniques for seagrass restoration are transplantation and seeding methods. Various seed-based planting techniques have been trialled around the globe, but the most successful large-scale restoration has been in Chesapeake Bay where 3600 ha seagrass has been restored (Orth et al., 2020). An interest in seagrass restoration in the UK has taken off in recent years with successful planting trials in Wales, using the seed-based BoSSLine method (Unsworth et al., 2019). The most appropriate restoration technique in Scotland will depend on the specific environment and local conditions of each proposed restoration site. Seagrass restoration research and activities are at an early stage in Scotland, however larger-scale projects such as Seawilding at Loch Craignish, the Solway Coast and Marine Pilot Project (SCAMPP), and multi-partner Restoration Forth have started, which will serve to increase the knowledge base. A seagrass restoration handbook and guidance has been developed for activities in Scotland to support these projects and ensure beneficial environmental outcomes (Kent et al., 2021). In addition, a marine and coastal enhancement project assessment framework for Scottish inshore waters has been produced (Chambers et al., 2022b). Focusing on four key habitats – native oyster, seagrass beds, saltmarshes and sand dunes – the framework provides overall guidance for enhancement projects including initial considerations and data requirements for licensing, advice on appropriate methods and approaches to minimise environmental and project risk.
Future research on seagrass beds
Whilst estimates of Scottish seagrass bed extent have improved in recent years, there are still a number of unmapped sites, with the potential for further unknown beds. Thus, a clear research gap is mapping Scottish seagrass adequately and reliably. This could potentially be achieved using a combination of satellite imagery, drone surveys, with ground-truthing by snorkelers. The effectiveness of drone surveys may depend on the site, as the delineation between habitats can be difficult to determine at some locations. Density and plant length also determine carbon standing stocks, but this aspect could be assessed during the ground-truthing process of the drone surveys.
Additionally, carbon sequestration rates are dominated by calculations based on two main species – P. oceanica and C. nodosa – with a regional bias (Fourqurean et al., 2012). Further research on Zostera spp. within Scottish meadows is required, to provide a more reliable estimate for the carbon sequestration rate. Scotland has dense Z. marina beds with high canopies, as well as smaller, patchy Z. noltii beds. How these differences potentially impact the blue carbon service should be explored through future research, especially as this may have implications for restoration from a blue carbon perspective. A recent PhD project ‘Understanding the drivers of carbon sequestration in Scottish seagrass’ at Edinburgh Napier University (Whitlock, 2022) has looked into three potential drivers of carbon sequestration in Scottish seagrass, specifically, decomposition, sediment characteristics, and rate of burial. Field data from eleven sites (ten intertidal meadows and one subtidal) across Scotland were collected and will contribute novel insights into what influences variability in seagrass carbon sequestration, which was seen between sites, and potentially between species.
Seasonal fluctuations in seagrass biomass production likely impact the blue carbon service. A study of Z. marina meadows in Sweden found a strong seasonal variation in carbon density and total sedimentary OC, with the former peaking in June (Dahl et al., 2020), likely coinciding with an increase in seagrass growth. Given that most studies to date have only sampled during the summer period, estimates of sedimentary carbon may not be representative of the yearly mean. Sampling could be repeated during more than one season to further clarify the impact of seasonality on Scottish seagrass carbon sequestration and storage. Improving carbon sequestration estimates will help clarify the importance of seagrass to Scottish blue carbon. Better understanding the drivers and threats may provide guidance on how to enhance potential stocks and determine optimum conditions for restoration from a blue carbon perspective.
Restoration of seagrass beds is gaining in popularity as a climate mitigation tool by increasing blue carbon stocks, as well as improving biodiversity. There is a Scottish specific research need to assess the feasibility of this approach and to quantify the additional blue carbon service restoration could potentially provide. As restoration work progresses, research should be targeted at comparing natural and restored seagrass beds with respect to carbon capture. While some work conducted in the USA has shown that restored seagrass beds can accumulate carbon at the same rates as natural beds (Greiner et al., 2013), this may not be representative of Scottish restored seagrass. Incorporating monitoring of carbon sequestration into restoration projects should therefore be a priority consideration for new restoration projects.
3.2 Kelp beds
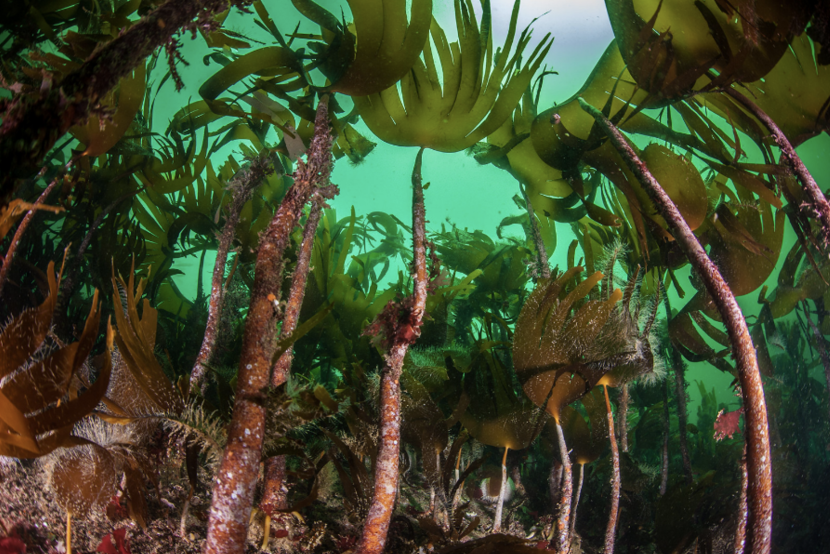
Kelp beds are dense macroalgae ecosystems that predominantly grow on hard or mixed sand and rock substrates, thus having little potential for local carbon sequestration (Krause-Jensen and Duarte, 2016; Macreadie et al., 2019). However, it has been suggested that these habitats contribute to blue carbon stores by acting as important carbon donors of allochthonous biomass to other systems, typically within sediments (Filbee-Dexter and Wernberg, 2020; Smale et al., 2018).
Extent of kelp beds
Utilising a combined approach of aerial photography and grab-sampling, Walker (1954) surveyed dominant kelp species (Laminaria spp.) along the Scottish coast during the years 1946-1953. Extrapolating the survey data to Scotland’s entire coast, an estimated 286,000 ha of kelp habitat was spread across the sublittoral (<19 m depth) area (Burrows et al., 2014; Walker, 1954). Efforts to map extent were made from the 1980s onwards by the JNCC’s Marine Nature Conservation Review (MNCR) using experienced survey divers (Burrows et al., 2014). Over 3,200 of these dives occurred in Scotland, extending into sea lochs such as Loch Ewe and Gairloch (Burrows et al., 2014). Burrows et al. (2014) proceeded to analyse the MNCR data utilising a statistical model to fit kelp abundance data, using various environmental variable predictors. A conservative estimate of 215,500 ha was calculated. This habitat suitability model was further developed in Burrows et al. (2018), to provide a fresh weight biomass for L. hyperborea of 19.73 Mt across the Scottish marine environment.
These are broad estimates with a range of associated caveats. The scale of the model influences the confidence in values. For instance, using large grid sizes when assessing the conditions suitable for kelp can lead to overestimations. Both modelling estimates assessed here use smaller grids (200 m2), which improves the accuracy of the model. In both cases, the model over predicts where there is insufficient underlying seabed substrate data, which poses another limitation. Any model is only as robust as its underlying data. The historical samples used to inform this model (MNCR surveys) have uncertainties of their own, which also impacts the confidence of any modelling outputs.
When using models to predict biomass or extent, arbitrary thresholds have to be set for observed and predicted density of plants and the predicted likelihood of finding kelp. Values are then reported as being the sum of areas above a certain threshold. In the case of the extent estimate (Burrows et al., 2014), it represents areas where kelp coverage is most likely to be at least Abundant – under the SACFOR scale, Abundant is defined as 20-40%. The biomass estimate (Burrows et al., 2018) represents P>0.5 of kelp being at least Rare, which is defined as coverage of 1-5% under the SACFOR scale. This highlights the issues that arise when attempting to compare estimates.
A more conservative value for biomass of L. hyperborea of 15.54 Mt may be more suitable, which represents where kelp biomass density exceeds 2 kg m-2 (Burrows et al., 2018). This corresponds to an area of 374,700 ha (Burrows et al., 2018). This value is higher than the previous modelling output (Burrows et al., 2014) and historical surveys (Walker, 1954). This discrepancy against historical surveys likely arises as Walker (1954) used spring grabs to survey, which is <100% efficient, resulting in an underestimate of kelp biomass.
Biomass scaling with a habitat suitability model should be constrained to predicting kelp biomass in areas of known kelp habitat, and a more conservative scaling factor should be adopted when attempting to estimate overall stock sizes over broader areas (Burrows et al., 2018). However, an overall value is needed to estimate subsequent blue carbon contributions of the Scottish kelp habitat. Thus, the lower biomass estimate of 15.54 Mt from the revised model (Burrows et al., 2018) will be taken forward, but the previously discussed caveats should be considered. There are also uncertainties associated with only using data from one kelp species, but as L. hyperborea is the dominant species within Scottish waters, this may not pose a high level of concern.
Blue carbon contributions from kelp beds
Burrows et al. (2014) reviewed the carbon standing stock in kelp beds within Scotland within the first national blue carbon audit. Utilising historic data (Kain, 1979; Walker and Richardson, 1955), total estimates of standing stock of Scottish kelp equated to 0.2 Mt C and 0.4 Mt C. This was based on area-specific standing crop values for Laminaria kelp of 94 g C m-2 (Walker and Richardson, 1955) and a revised, higher value of 187 g C m-2 (based on Kain, 1979) respectively, using weight conversion assumptions from Krumhansl and Scheibling (2011). The value was revised as Walker and Richardson (1955) had used spring grabs to survey, which is likely <100% efficient, resulting in an underestimate of kelp biomass. Kain (1979) used samples collected by divers, which is a more efficient method. However, these samples were restricted to the shallowest depths of maximum abundance, rather than the wider 0-9 m range sampled by Walker and Richardson (1955). Standing stock diminishes rapidly with depth, with the rate of decline being site dependent – faster where light attenuation is more rapid and least in clearer water (Burrows et al., 2014). Therefore, Kain’s (1979) original average area-specific standing stock estimate of 652 g C m-2 may be an over representation.
More recently, Pessarrodona et al. (2018) found a mean (± SE) standing stock of 972.2 ± 41.1 g C m-2 for kelp beds near Stromness and Oban. This is agreeable with the standing stock presented graphically from another study with Scottish sites (Smale et al., 2020). Standing stock was also assessed for L. digitata in Scotland, finding values of 278 and 166.9 g C m-2 in the peak and reduced growth season respectively (King et al., 2020). However, surveys were again only conducted down to a maximum of 7m depth, so the larger figures likely represent an overestimate if applied across all kelp bed sites within Scotland. Additionally, these localised surveys may not be representative of the wider Scottish environment.
Utilising the lower biomass estimate of 15.54 Mt (or 374,700 ha) for L. hyperborea described in the previous section (Burrows et al., 2018), combined with the more recent, lower area-specific stock of 166.9 g C m-2 (King et al., 2020), provides a standing stock estimate for Scottish Laminaria kelp of 0.6 Mt C. This relies on the underlying assumptions adopted by Burrows et al. (2014) whereby dry weight is 15% of fresh weight, with a 30% carbon content of dry matter (from Krumhansl and Scheibling, 2011). However, these conversions are based upon the kelp species Saccharina longicruris and L. digitata from Nova Scotian kelp beds (Krumhansl and Scheibling, 2011). Applying these percentages to Scottish kelp beds, which are dominated by L. hyperborea, leads to further uncertainties arising from potential regional and species differences.
Global rates of net production for typical UK kelp species (Laminaria and Saccharina) range from 120 g C m-2 yr-1 in Loch Creran, Scotland, to 1938 g C m-2 yr-1 in Rhode Island, USA (Burrows et al., 2014). The average global rate of net production reported by Burrows et al. (2014) was 685 g C m-2 yr-1, with a large standard deviation of 656 g C m-2 yr-1. A more recent study (Smale et al., 2020), focusing on L. hyperborea in the Northeast Atlantic, found an average net primary productivity of 340 g C m-2 yr-1, with a range of 166 to 738 g C m-2 yr-1. Looking at just studies in Scotland, an average of 378 g C m-2 yr-1 can be reported (Smale et al., 2020). This estimate carries a high degree of uncertainty, resulting from: the use of differing methods; habitat and depth-specific rates; and varying environmental factors (light availability, nutrients, temperature). This value is likely to be an overestimate, given that the majority of research was based on shallow water estimates, with all studies occurring at <15m. Combining the average production rate for kelp in Scotland, with the habitat extent of 374,700 ha, which represents areas where kelp biomass density exceeds 2 kg m-2 (Burrows et al., 2018), gives a total estimated net production capacity from kelp in Scottish waters of 1.4 Mt C yr-1.
Fate of kelp detritus and potential for carbon sequestration
On average, kelp forests export approximately 80% of their production (Krumhansl and Scheibling, 2012), with a recent study across UK kelp beds reporting export rates >98% from six out of eight sites (Smale et al., 2022). Detritus is formed through the dislodgment of whole kelp plants and the loss of lamina tissue through erosion or May cast (Pessarrodona et al., 2018). May cast is a term that refers to the major detrital pulse occurring after the shedding of the prior season’s lamina growth, which remains attached to the newly growing lamina until it is lost entirely between March and May. This detritus supports marine food webs, and may ultimately be transported across the open and the deep ocean, where a significant proportion is sequestered in sediments (Krause-Jensen and Duarte, 2016; Ortega et al., 2019). A proportion of this detritus is also washed onto the beaches of Scotland throughout the year, known as beach-cast (Orr, 2013). It then acts to support coastal food webs, most notably higher trophic levels including wader species (Orr, 2013).
In general, the slower the decomposition of kelp detritus in the ocean, the greater chance for sequestration in the deep ocean, thus taking longer to release CO2 to the atmosphere (Lutz et al., 2002; Ortega et al., 2019). Macroalgae detritus that reaches open ocean depths below 1000m is considered trapped in water masses, where the CO2 is retained for >1000 years, before returning to the ocean surface and eventually entering the atmosphere (Krause-Jensen and Duarte, 2016; Ortega et al., 2019). Additionally, detritus that is retained in deep fjords or basins with high rates of sedimentation may also be buried between 100 and 1000 years (Filbee-Dexter et al., 2018; Filbee-Dexter et al., 2020). Krause-Jensen and Duarte (2016) estimated that on average, 11.37% of global net primary production from macroalgae is sequestered and incorporated into long-term storage. There are many uncertainties associated with this flux, but applying this proportion to the estimated kelp production capacity, suggests that 0.27 Mt C yr-1 could be sequestered from Scottish kelp.
Threats to kelp beds
Kelp beds and kelp communities on sublittoral sediment are both considered PMFs in Scotland, with beds protected by a suite of MPAs. Kelp forests (analogous to kelp beds) are a recent addition to the OSPAR list of Threatened and Declining species because of significant declines within the OSPAR maritime area. Threats to kelp beds have been reviewed by Smale et al. (2013), with a particular focus on the coasts of the UK and Ireland. The main threats identified were climate-driven pressures; increased turbidity and nutrient input from industrial runoff; removal of top predators leading to overgrazing of kelp beds; and unsustainable harvesting.
Harvesting may be less of a threat in Scotland because it is a regulated activity. Revised regulations permit sustainable harvesting limits, whilst safeguarding the functions and services that kelp habitats provide. The risks associated with large-scale, industrial wild kelp harvesting in the UK have diminished since the enactment of Section 15 of the Scottish Crown Estate Act 2019, which includes restrictions on the removal of wild kelp from the seabed and its use for commercial purposes.
Certain species of kelp are sensitive to temperature. The geographic range of Saccorhiza polyschides, a prevalent UK species, has undergone range contractions and declines in abundance, due to increases in seawater temperature (Fernández, 2011). In another study, carbon assimilation in multiple kelp forests across the UK coast along a latitudinal gradient was compared (Pessarrodona et al., 2018). The rocky reef sites were comparable in terms of key environmental variables, however, thermal regimes varied, with a total 2°C difference between the north of Scotland and the south of England sites. It was found that kelp forests within the warmer climatic regimes assimilated, on average, at least three times less carbon and donated less than half the amount of particulate carbon compared to those habitats in cold regimes (Pessarrodona et al., 2018). Recently, a significant relationship has been highlighted between temperature and kelp detritus decomposition rates across the northern hemisphere, with markedly slower decomposition in cooler northern regions relative to warmer southern regions (Filbee-Dexter et al., 2020). Thus, increasing seawater temperatures associated with climate change may have implications for both kelp species abundance and distribution, as well as their potential contribution to carbon sequestration.
In conjunction with ocean warming, increased storm severity and frequency will also impact kelp habitats (Smale et al., 2013), damaging plants and changing dynamics of whole beds. Coastal runoff of sediment and nutrients is also likely to negatively impact macroalgae habitats, as this reduces water clarity, resulting in increased light attenuation (Burrows et al., 2014), but this is likely not a significant issue for Scotland’s kelp. In the UK, overgrazing of macroalgae habitats by urchin is generally restricted and patchy, but this may be a concern to kelp beds from a global perspective (Smale et al., 2013).
Future research on kelp beds
There are a number of knowledge gaps in the role of macroalgae in carbon sequestration. Firstly, standing stock values should be calculated for Scottish kelp species across their full depth range. The limited productivity data collected within easily accessible shallow depths likely biases the production rates, and the overall carbon standing stocks calculated from these. Going forward, kelp sampling should include deeper beds to create a more balanced picture. Further research should also aim to improve estimations for the carbon content of kelp within the Scottish environment, at a range of locations, and within different seasons.
The statistical model used in estimating kelp biomass utilises satellite-derived chlorophyll a concentrations, which appears to be a fairly robust method. Though the caveats discussed previously should be considered, with confidence assigned to the estimates accordingly. There is a need for a scoping study to assess the current extent of Scottish kelp beds and determine if this is agreeable with the modelling estimate, which is largely based on data collected prior to 2000, with a range of modelling uncertainties. Mapping kelp extent would be costly and require large effort, although approaches using satellite imagery, drones or other remote sensing techniques may progress this work. However, the application of these methods in this context is still in development.
The main challenge however remains tracing and understanding the large export flux from productive coastal habitats to the open ocean (Krause-Jensen et al., 2018). Accounting for the fate of this high production and determining the proportion that is sequestered over the long-term, is imperative in assessing the contribution of macroalgae to Scotland’s blue carbon.
4. Calcifying aggregations
Habitats dominated by calcifying organisms are essential for a biodiverse, healthily functioning marine ecosystem and provide multiple stacked benefits (zu Ermgassen et al., 2020). For instance, they contribute to climate change adaptation through wave energy dissipation and provide essential fish habitat (Kent et al., 2016). In addition, these habitats contribute to long-term stores (>100 years) of inorganic carbon (carbon comprises 12% of the carbonate molecule) and make an important contribution to the overall carbon budget (e.g., Porter et al., 2020). They potentially also play a supporting role in climate change mitigation through the facilitated biodeposition of organic carbon into underlying sediments (Lee et al., 2021; Lovelock and Duarte, 2019).
However, the process of calcification (the production of the inorganic carbon that forms the basis of these habitats) results in a net release of CO2 (Frankignoulle and Gattuso, 1994; Macreadie et al., 2017). Further evidence is required to quantify the relative balance between CO2 release during calcification (source) and any accumulation either during burial of organic matter (sink) (van der Heijden and Kamenos, 2015), or, in relation to photosynthesising organisms such as maerl, the additional contribution of photosynthesis, to understand the net effect. Disturbance of these two carbon forms have contrasting implications for climate too, with the breakdown of organic matter potentially leading to a release of CO2 (Turrell et al., In Review).Therefore, due to the unknown net balance of carbon dioxide, inorganic carbon accumulation by calcifying aggregations cannot be considered to have the same climate mitigation potential as vegetated blue carbon
The description used by Holt et al. (1998) to describe biogenic reefs has been modified for this review to reflect the inclusion of further habitats, grouping them under the novel term calcifying aggregations. The adopted definition for calcifying aggregations is as follows –
Structures which are created by accumulations of organisms, clearly forming a substantial, discrete community or habitat that is very different from the surrounding sea bed. The structure may be composed almost entirely from the organism and its calcium carbonate tubes, shells or skeletons, or it may to some degree be composed of sediments, stones and shells bound together by the organisms.
This definition encompasses a range of habitats, which include –
- Maerl beds
- Cold-water coral reefs
- Serpulid aggregations (Serpula vermicularis)
- Flame shell (Limaria hians)
- Horse mussel beds (Modiolus modiolus)
- Blue mussel beds (Mytilus edulis)
- Native oyster (Ostrea edulis)
- Brittlestar beds (Ophothrix fragilis)
- Bryozoan thickets (Flustra foliacea)
In the literature, most of the habitats discussed in this section are considered biogenic reefs, using the original definition by Holt et al. (1998). This definition would encompass cold-water coral, serpulid, flame shell, horse mussel, and blue mussel habitats, with this grouping often present in the literature (Burrows et al., 2014, 2017). However, it does not include benthic habitats such as maerl beds, brittlestar beds or bryozoan communities. These deposits represent substantial concentrations of benthic biomass and they all build tubes, shells or skeletons from calcium carbonate (CaCO3), which may persist on the seafloor or become incorporated into bottom sediments following death as shell material (Burrows et al., 2014). They can also trap associated biota in their matrix-like structures, further increasing their contribution to organic carbon storage. As these benthic habitats all have similar underlying mechanisms contributing to how they store carbon, predominantly in the inorganic form, this review has grouped them under the term calcifying aggregations.
This section will review the literature currently available to calculate inorganic carbon standing stocks (and organic values where appropriate), report IC production rates, and will attempt to calculate the potential annual IC production capacity, for each habitat, using Scottish habitat extent values.
4.1 MaxEnt Modelling
“MaxEnt” modelling is used by researchers as a method to predict habitat suitability (and therefore potential habitat extents) from incomplete or a paucity of sample data (Gormley et al., 2013). The habitat extent values for a number of the calcifying aggregation habitats within this section have been informed to some degree by predictive habitat suitability models, and therefore have a relatively high level of uncertainty. MaxEnt, which stands for maximum entropy modelling, enables the modelling of species distributions from presence-only species records. This is achieved through predicting habitat suitability using environmental variables in order to estimate the potential geographic distribution (Elith et al., 2011). MaxEnt typically outperforms other methods based on predictive accuracy (Elith et al., 2011). However, the properties of MaxEnt models have several implications. Models that rely on presence-only data are more affected by sample selection bias (relating to uneven sampling intensity), which may reduce confidence in its outputs (Elith et al., 2011). Additionally, predictions of habitat suitability from environmental layers are restricted by sample size with limited data meaning that the full ecological niche of a species/habitat may not be realised (Elith et al., 2011). Multiple habitats can naturally exist as a mosaic at the same location and accordingly will have less than 100% coverage in such a situation. This is not necessarily considered within MaxEnt models and a consequence of this could be “double-counting” of habitats predicted at overlapping locations, in effect, over-estimating habitat extent. As with any modelling prediction, the confidence in its outputs is only as high as the underlying data. Whilst MaxEnt modelling may be a useful tool in providing first-order estimates of extent, additional ground-truthing or scoping studies should be conducted where possible to inform any predictions. These limitations should be considered when reviewing modelling based predictions and taken into account for the following section.
4.2 Maerl beds
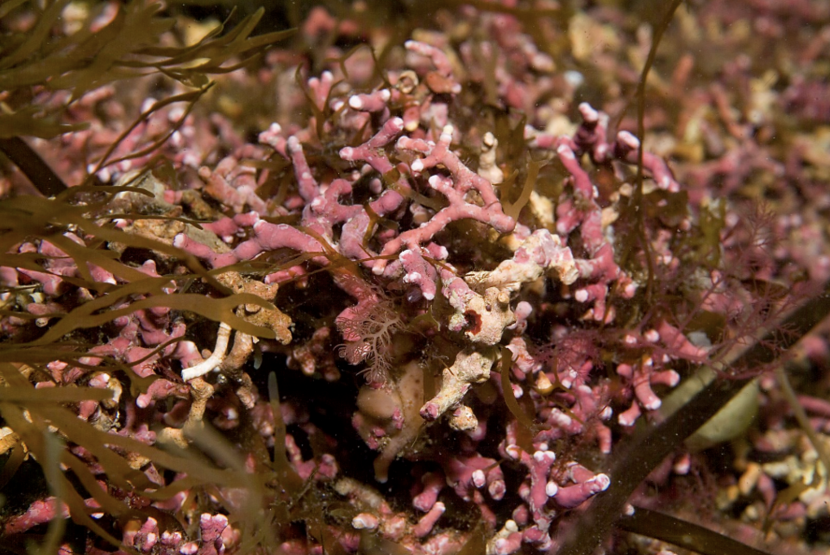
Maerl refers to live and dead accumulations of red coralline algae, which form calcium carbonate skeletons. They grow as unattached nodules, with complex branching structures, forming extensive beds (Burrows et al., 2017). Living maerl is restricted to the surface layer of the beds, overlying the chalky skeletons of dead maerl that has accumulated over centuries. The proportion of live to dead maerl is variable across sites, with accumulations considered a bed where there is at least 20% coverage of dead or live maerl thalli (SNH, 2018a). Coralline algae can act as both CO2 sinks, through photosynthesis and CaCO3 dissolution, and CO2 sources, resulting from respiration and CaCO3 production (van der Heijden and Kamenos, 2015). With carbonate production rates similar to that of OC production within mangroves, saltmarshes and seagrasses (van der Heijden and Kamenos, 2015), coralline algae represents a significant inorganic carbon store globally.
Distribution and extent of maerl beds
Typical species found in Scottish waters include Phymatolithon spp. and Lithothamnion spp. (Birkett et al., 1998). In Scotland, maerl is extensive along the west coast, and in the Western Isles, Orkney and Shetland (Hall-Spencer et al., 2008). Burrows et al. (2014) provided a rough estimation of total Scottish extent of 706 ha based on multiplying the number of maerl bed records (353) from GeMS by an assumed bed area of 2 ha. Further work has since been conducted to develop polygon data for Scottish maerl beds. Taking recent data from GeMS V8_i24 (accessed Jan 2021), known maerl extent in Scotland is estimated to be 3,143 ha from 527 records.
However, a recent MaxEnt modelling study (Simon-Nutbrown et al., 2020) utilising occurrence points from GeMS, predicted the total area suitable for coralline algal bed presence to be 713,000 ha in Scotland. Some areas of predicted distribution were not covered by GeMS presence records. Field validation of two positive prediction sites were found to be true and one modelled absence site was confirmed to not have any free-living coralline algae present (Simon-Nutbrown et al., 2020). Whilst these validations corroborate the reliability of the model, further ground-truthing would be required before applying the modelled maerl extent estimate. Given the large disparity between this modelling prediction and the recent estimate from GeMS, the latter conservative value will be used to assess the Scottish blue carbon contribution. However, the modelled extent highlights the uncertainty in this parameter and the likelihood that there are undiscovered maerl beds or large areas of unmapped beds.
Blue carbon contribution from maerl beds
Very little information is available in regards to maerl (alive or dead) deposit thickness, although beds in the Wyre sound were reported to have a mean thickness of 1.175 m (Porter et al., 2020). On average, 0.6 m thickness is used for calculations, as this is based on various observations (Burrows et al., 2014). This average could not be corroborated through assessing maerl bed records on Marine Recorder, as information on bed thickness was rarely documented. Assuming maerl has a density of 0.8667 t m-3, with 12% of constituent calcite as IC, this gives a standing stock per unit area, or density, of 624 t IC ha-1 for an average thickness of 0.6 m (Burrows et al., 2014). This equates to 104 t IC ha-1 for 0.1 m, an often-used depth standard. However, the density of material is not homogenous within many calcifying habitats and standardised values, whilst being useful for general comparisons, should be used cautiously. Multiplying the density by the total extent of 3,143 ha for the average bed thickness of 0.6 m, gives an estimated IC standing stock of 1.96 Mt within Scottish maerl deposits. This value likely represents an underestimate, as there are likely undiscovered maerl beds and likely variations in bed thickness across sites. Additionally, the estimate does not include the dead maerl that forms beach sediment (e.g. Plockton, Isle of Skye, Outer Hebrides), again leading to an underrepresentation of total IC stored (Burrows et al., 2014).
A recent study (Mao et al., 2020) assessed the total OC deposited within cores of a maerl bed along the west coast of Scotland. A value of 12.28 t OC ha-1 was determined for this particular site. Multiplying this estimate by the conservative value for extent (Burrows et al., 2014), gives a total OC value of 38,600 t stored within Scottish maerl beds, highlighting the role of maerl as a supporting blue carbon habitat. Further studies are required to increase the confidence of this estimate.
There is sparse information relating to the live or dead status of various maerl habitats in Scotland, but through various observations, beds range from 100% live down to <5% live (Burrows et al., 2014). Previously a blanket average of 50% live was adopted as a rough estimate (Burrows et al., 2014). However, there are large uncertainties associated with this value. To improve upon this estimate, >1,700 Scottish maerl records from Marine Recorder were assessed for mention of the live or dead status. Only records that clearly stated a percentage of live or dead were used. An average of 103 records found a value of ~30% live. This does not include entries with qualitative comments on bed status, as the interpretation of these statements likely vary between different surveyors.
Previous reviews have opted to use carbonate production rates for L. glaciale and P. calcareum from studies in Norway and Ireland respectively, as no Scottish specific estimates exist (Burrows et al., 2014; 2017). Based on three European studies, carbonate production rates ranged from 29 - 1,432 g CaCO3 m-2 yr-1 (Bosence & Wilson, 2003; Martin et al., 2007; Savini et al., 2012), depending on species. Using these values, a mean carbonate production rate of 477.9 g CaCO3 m-2 yr-1, or 57.4 g IC m-2 yr-1 is estimated. Applying the broad average of 30% live beds, and multiplying by the value of Scottish extent, provides an average for the total carbonate production capacity of 4,506 t CaCO3 yr-1, equivalent to approximately 541 t IC yr-1.
Threats to maerl beds
Maerl beds are considered to be marine conservation priority habitats; as an OSPAR threatened and/or declining habitat, a PMF in Scotland, as a protected feature within MPAs and are protected as a component of sandbank or large shallow bays habitats within Special Areas of Conservation (SACs). Evidence of deterioration in the condition of maerl beds in the Wester Ross MPA and South Arran MPA led to a ‘recover’ conservation objective being set for the feature at both sites (SNH, 2018a).
There are various threats to maerl beds and their capacity to sequester carbon. Maerl beds are highly sensitive to physical disturbance, particularly in terms of abrasion, habitat removal, organic enrichment, siltation and changes in water flow (Hall-Spencer and Moore, 2000a; Perry and Tyler-Walters, 2018). One pass of a scallop dredge led to 70% of live maerl present being buried and subsequently dying, with no sign of recovery during a four year monitoring period (Hall-Spencer and Moore, 2000a). Physical disturbance may also release carbon associated with these deposits (Burrows et al., 2014). Interactions with finfish aquaculture also threatens maerl beds, with organic fish farm waste shown to reduce both live maerl cover and infaunal species biodiversity (Hall-Spencer et al., 2006; Haskoning UK Ltd., 2006). Where live maerl persists following disturbances, their incredibly slow growth rates (e.g. 0.1-1 mm yr-1 - Lancaster et al., 2014; Mazik et al., 2015) hamper the recoverability of the habitat. This further increases their sensitivity to pressures.
Additionally, climate-driven pressures will threaten maerl over the long-term. An increase in benthic temperature was found to be a significant driver in predicted maerl loss (Simon-Nutbrown et al., 2020), using modelling predictions based on Representative Concentration Pathways (RCP)(IPCC, 2014). The business as usual RCP 8.5 scenario predicted an 84% decline in habitat coverage by 2100. Even under the very stringent RCP 2.6 pathway, predicted losses of 63% were still expected to occur by 2100. The impacts of an increase in temperature may be further amplified by the effects of ocean acidification (Martin and Hall-Spencer, 2017), as a lower pH can lead to structurally weakened maerl (Kamenos et al., 2013). These climate-driven pressures will likely prevent maerl growth, halting the carbon sequestration. Over longer timescales, ocean acidification may also lead to the chemical and physical breakdown of maerl deposits, releasing the carbon they hold (Burrows et al., 2014). Elevated temperatures may also enable the spread of invasive non-native species (INNS) (Occhipinti-Ambrogi, 2007). The invasive American slipper limpet, Crepidula fornicata, is known to cause smothering of subtidal habitats and is currently a concern to maerl beds in Pembrokeshire and Falmouth (Allen et al., 2014; Pembrokeshire Marine Special Area of Conservation, 2008). It is important that risks posed by INNS now and in the future are evaluated and appropriate biosecurity implemented for the benefit of vulnerable habitats such as maerl. Climate-driven pressures are additive and will exacerbate the impacts from other anthropogenic pressures.
Future research on maerl beds
Whilst Scottish maerl may not have a high carbonate production capacity, this review highlights the potential value of maerl beds to Scottish blue carbon deposits. Their high sensitivity and slow growth rates highlight the need to protect known maerl beds by reducing pressures such as introducing fisheries management measures. Management guidance will be discussed further in the subsequent evidence map.
There are large uncertainties in the various calculations of blue carbon contributions. Results presented here are likely underestimates – the extent of many maerl beds is unknown and there may be additional undiscovered sites. Further efforts should be focused on mapping and ground-truthing the modelling predictions of Simon-Nutbrown et al. (2020). Furthermore, the dead maerl that forms sediment along certain Scottish beaches has not been accounted for in these estimates. Another issue arises from estimating bed thickness and the variable proportion of live to dead maerl, as these values have not been widely measured. Bed thickness is a difficult feature to incorporate into recording processes, as depth estimates require core sampling. Inclusion of this consideration in future sampling and monitoring of maerl beds would be welcome to improve confidence in estimates for bed thickness.
4.3 Cold-water coral reefs
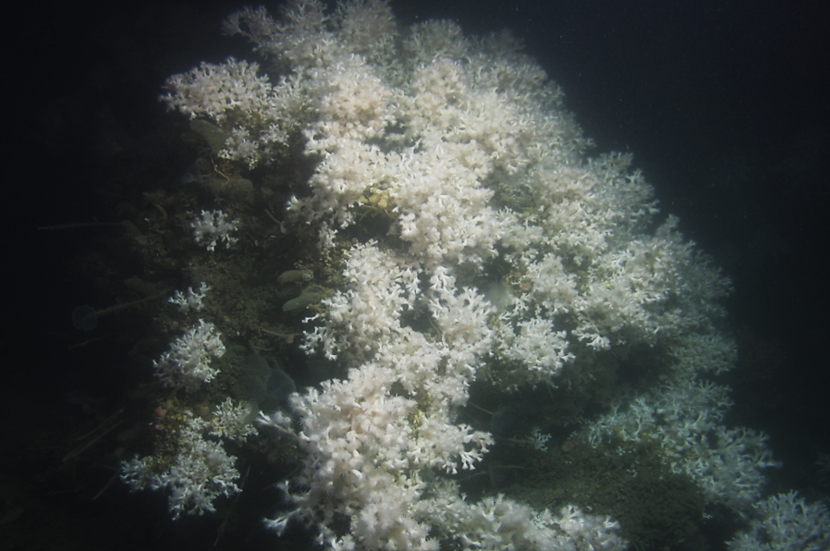
The cold-water coral, Lophelia pertusa, forms patches of bushy growths, growing into thickets and eventually forming reefs made up of hard skeleton (Garrard et al., 2019). Reefs can exist for thousands of years and are structurally–complex supporting biodiversity, for instance through provision of essential fish habitat (Roberts et al., 2005). Cold-water corals can grow in deep (> 100 m) and dark waters. There are a Priority Marine Feature within Scottish waters (SNH, 2018e).
Distribution and extent of cold-water coral reefs
Cold water coral reefs are found at depths too deep to be surveyed using satellite-based remote sensing techniques making surveying challenging (Roberts et al., 2005). Lophelia pertusa is widely distributed along the north-east Atlantic margin, with most records found in the offshore banks at a depth range of 100-1500 m (Roberts et al., 2003; 2005). In Scottish waters, there are numerous historical records from the offshore banks, ridges and seamounts of the Rockall Trough, as well as localities around the Shetland Islands, along Wyville-Thomson Ridge and on Hatton Bank (Roberts et al., 2003). More recently, Ross & Howell (2013) trialled MaxEnt modelling methods to predict suitable habitat extent within the NE Atlantic, with model performance ranging from fair to excellent. The coarse resolution of the model variables likely precluded the detection of fine-scale mesohabitats <750 m in size, highlighting areas for improvement. Due to the vast spatial extent and limited resources available for offshore habitat mapping, little is still known about the coral reef extent at these sites (Burrows et al., 2014).
There are currently two well-defined coral reefs within Scottish waters – the Darwin Mounds and the Mingulay Reef Complex – both of which are designated as marine Special Areas of Conservation (SACs) (Roberts et al., 2005; Burrows et al., 2014Burrows et al., 2014). The Darwin Mounds SAC is estimated to cover a total area of 140,000 ha, which includes two main concentrations of mounds (De Santo, 2013), covering an area of 144 ha (Burrows et al., 2014). Total area of cold-water coral reefs for the Mingulay Reef Complex is estimated to be 540 ha (Davies et al., 2009). Combined, these reef complexes span approximately 684 ha, with a likely significant but unknown total volume. For instance, live coral on the ridge at the Mingulay Reef Complex can be found between 120 and 190 m depth (De Clippele et al., 2021).
Cold-water coral reefs are also known to form on anthropogenic structures, such as offshore oil and gas platforms (Roberts, 2002; Gass and Roberts, 2006; Macreadie et al., 2011). Reefs have been identified on 13 of 14 platforms examined using existing oil and gas industry visual inspections (Gass and Roberts, 2006). However, no attempt has been made to fully quantify their extent or determine how they may differ from natural formations.
Blue carbon contributions from cold-water coral reefs
To determine a mass-volume relationship for L. pertusa, Burrows et al. (2014) assembled 13 coral blocks collected west of Shetland into a structure measuring 85 x 100 x 20 cm, filling a total volume of 0.17 m3 and weighing 53 kg. A mass-volume relationship of 312 kg m-3 was thus estimated (Burrows et al., 2014). This figure may not be representative of diffuse-branching colony morphs as the coral specimens used were of the domed, high-density morphotype, and caution should be applied. Using the mass-volume relationship above, an IC density of 374 t IC ha-1 is calculated by accounting for calcite, comprising 12% of the calcium carbonate structure, and converting to a hectare metre (an area of a hectare with a 1 m depth). This is equivalent to a crude standardised IC density of 37.4 t IC ha-1 for 0.1 m depth. Utilising the estimates for distribution and taking into account mound diameter, number of colonies, and the distance between colonies, the total standing stock of inorganic carbon for the Darwin Mounds is approximately 13,500 t (Burrows et al., 2014). Using conservative estimates of coral volume and treating the mounds as regular cones, gave a total standing stock of carbon for the Mingulay Reef Complex of approximately 112,000 t (Burrows et al., 2014). A combined total standing stock of 125,500 t IC is thus estimated.
More recently in a study that successfully produced reef-scale biomass maps at the Mingulay Reef Complex, De Clippele et al. (2021) estimated the average live mass (skeletal weight and biomass) of a L. pertusa colony to be 0.74 kg m-2 (range 0.09-1.66 kg m-2) containing organic and inorganic carbon. The average dead skeletal weight of a L. pertusa colony being 1.6 kg m-2 of presumably carbonate material (range 0.09-1.66 kg m-2). These data were used to estimate a total mean of 126 t C and 339 t C stored within the live and dead L. pertusa framework, respectively, for the total Lophelia reef habitat area of 170 ha. It is not immediately clear how this carbon mass is split into organic and inorganic fractions however.
Studies of this nature will help to strengthen estimates of total carbon stocks and turnover, and our understanding of cold-water corals as a net source or sink of carbon (De Clippele et al., 2021). Such few studies currently however, highlights the need for a consistent approach to reporting parameters, such as area/density, biomass, and carbon metrics (e.g. mass, composition of OC/IC, turnover, production etc.) for reef habitats making comparison of datasets difficult. For the purposes of this review we have used the standing stocks calculated above for the combined Darwin and Mingulay Reef complexes, although acknowledge the large uncertainties associated with these and requirement for further data.
Radiometric dating of samples from Mingulay provided a mean growth rate of 3-4 mm yr-1 (Douarin et al., 2014), with a peak of 12 mm yr-1. Reef growth has varied during the late Holocene, likely sensitive to shifts in North Atlantic circulation. The current growth rate is at the slower end of the spectrum, despite conditions being conducive for coral growth. Converting the average figure into a carbonate production rate results in a rate of approximately 294 g CaCO3 m-2 yr-1 (Burrows et al., 2014). This represents an equivalent net IC production rate of 35 g IC m-2 yr-1 (Burrows et al., 2014). Assuming the same production rate for the Darwin Mounds, the total net inorganic carbon production capacity of both reefs is 239.4 t IC yr-1. However, the sequestration rate is likely lower for the Darwin Mounds, as these colonies grow in deeper, less productive environments (Burrows et al., 2014), thus the total sequestration rate quoted may be an overestimate.
Threats to cold-water coral reefs
Cold-water coral reefs are considered to be marine conservation priority habitats; as an OSPAR threatened and/or declining habitat, a PMF in Scotland, as well as being on the Scottish Biodiversity List, a list comprising species and habitats of principal importance for biodiversity conservation. Cold-water coral reefs are a protected feature within a number of deep sea MPAs off the west coast of Scotland including the East Mingulay, Darwin Mounds, North-West Rockall Bank Special Areas of Conservation (SAC) and the West of Scotland MPA.
A review by Hall-Spencer and Stehfest (2009) summarised the threats to L. pertusa. Fishing was determined as the main pressure, as the use of bottom-contact gear can lead to physical damage to the habitat (Hourigan, 2009). As a result, a ban on trawling within the Darwin Mounds SAC was made permanent in 2004 (De Santo and Jones, 2007). Follow-up surveys several years after designation to assess the impact of the trawling ban have concluded little to no recovery on the parts of the reef that incurred significant damage from fisheries (Huvenne et al., 2016). However, healthy coral growth was seen in areas that had previously suffered minimal damage highlighting that marine conservation measures in deep-sea environments could be effective, as long as they were implemented before damage occurs because of the slow recovery times for deep-sea ecosystems (Huvenne et al., 2016). East Mingulay SAC also has fishing restrictions in place for both mobile gear and creeling implemented through The Inshore Fishing – Prohibition of Fishing and Fishing Methods (Scotland) Order 2015. In addition to direct impacts, L.pertusa reefs are sensitive to changes in suspended solids and increased turbidity (NatureScot, 2019). Growth rates of this species have been shown to decrease under elevated CO2 concentrations expected with ocean acidification (Büscher et al., 2017). Research has also shown an impact of ocean acidification on cold-water coral skeletal porosity, resulting in a lower complexity of coral reefs (Hennige et al., 2020). This may further exacerbate how this cold-water coral is able to respond to different environmental pressures.
Future research on cold-water coral reefs
Whilst current extent data are limited for L. pertusa reefs in Scotland, increasing the mapping effort from a blue carbon perspective is not considered a high priority. Cold-water coral appears to have a relatively low carbonate production rate (e.g., Douarin et al., 2014) and a modest carbon density compared to other habitats (Figure 3). Efforts would be better placed in conservation management to reduce managed pressures on these habitats to enhance biodiversity and associated ecosystem services, including blue carbon contributions (Jackson et al., 2014). This would help to ensure that reefs are more resilient to the likes of climate change. For instance, the cumulative impacts of unmanaged pressures, such as ocean acidification, can result in ecosystem-habitat scale loss (Hennige et al., 2020), and therefore should be considered within marine management decisions.
Cold-water coral is also known to grow on anthropogenic structures including oil platforms, however current decommissioning regulations to remove infrastructure at the end of operational life suggests this is a temporary contribution to any blue carbon service. A research project, INSITE, is underway to look at the disturbance impact of decommissioning to the blue carbon service provided by sediments; however, this does not extend to in-situ habitats on the infrastructure. This could warrant further investigation to improve understanding of the blue carbon contributions of such habitats, however it is not expected to be significant.
Additional measurements of carbonate production rates for deeper, less productive sites such as the reefs of the Darwin Mounds, would improve stock estimates. However, as production rates are generally low in comparison to other blue carbon habitats, this evidence gap is not recommended to be a priority and instead should serve as a caveat when reporting carbonate production rates.
4.4 Serpulid aggregations (Serpula vermicularis)
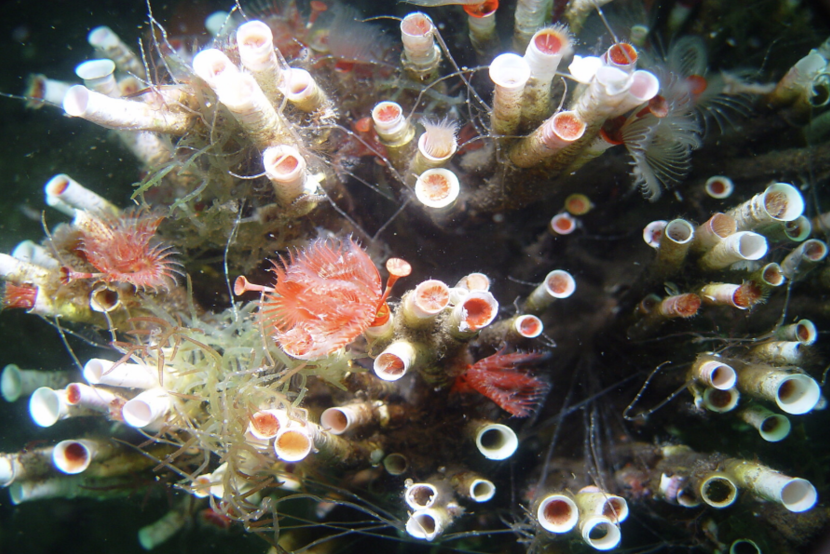
A serpulid aggregation consists of dense clumps of white chalky tubes, each containing a Serpula vermicularis worm (SNH, 2018b). Individual tubes can grow to 5 mm in width and 15 cm in height. The aggregations can extend to 1 m in height and 2 m wide by growing on top of each other on stones or shells in muddy sediments.
Distribution and extent of serpulid aggregations
The serpulid polychaete Serpula vermicularis is common and widespread in Scottish waters, but reef-forming aggregations are restricted to only a few sites (Burrows et al., 2014). These Scottish sites have previously included Loch Creran (Moore et al., 1998), Loch Teacuis (Dodd et al., 2009) and Loch Ailort (SNH, 2018b). Serpulid aggregations were also present in Loch Sween during the 1980s, but have since disappeared (Hughes et al., 2008). The extent of the habitat within Loch Creran and Loch Teacuis has declined by approximately 20% between 2005-2019 with widespread fragmentation and reduction in tube occupancy reported from surveys (SNH, 2015; Tulbure, 2015; Moore et al., 2020). Repeat monitoring carried out in March 2023 at Loch Ailort failed to identify any evidence of live or dead serpulid aggregations in the location of the baseline diver transects that were established in 2017 (Sayer-Mitchell et al., 2023).
The largest extent of these known aggregations occurred in Loch Creran where it was reported to cover 108 ha (Moore et al., 2009). This is agreeable with data from GeMS V8_i24 (accessed Jan 2021), which records an extent of around 97 ha, though this is based on survey data from 1998. Percentage cover of serpulids at this site ranged from 4.86-16.88%, with a mean value of 11.23% coverage, along four transects surveyed by divers (Moore et al., 2009). Taking this mean estimate, the total serpulid area for Loch Creran is estimated to be 12.1 ha. Serpulid aggregations of Serpulid aggregations of Loch Teacuis occupy a narrower band, with a habitat coverage estimated at 20 ha (Dodd et al., 2009) within which reef coverage was approximately 4% equating to 0.8 ha (Dodd et al., 2009). A NatureScot survey in 2015 concluded that the living reefs were largely absent apart from scattered fragments and a few aggregations on artificial structures (Kamphausen et al., 2015), and a further survey in 2022 showed no improvement. Estimates of coverage are not known for Loch Ailort, but are likely less than total serpulid area for Loch Creran. Total known Scottish extent is estimated to be 12.9 ha. However, as this figure does not the reported declines in extent seen in Loch Creran (up to 20%) (Moore et al., 2020) or Loch Teacuis, this is likely to be an overestimate for serpulid reef coverage (SMA, 2020a).
Blue carbon contributions from serpulid aggregations
Burrows et al. (2014) determined the mass-volume of an improvised serpulid reef using fragments of tubes taken from Loch Creran; a value of 28,644 g CaCO3 m-2 was estimated for clusters approximately 0.3 m in height, equating to 3,437.3 g IC m-2 or 34.37 t ha-1 IC. Utilising this estimate and that of habitat extent above, the carbonate standing stock for serpulid reefs was estimated at 6,931 t CaCO3 for Loch Creran and 229 t CaCO3 for Loch Teacuis (Burrows et al., 2014). Combined, this is a total standing stock of 7,160 t CaCO3, equating to 859 t IC (12%). These figures were based upon measurements from tube clusters that were mostly 0.2-0.3 m tall, which is a reasonable estimate for Loch Teacuis based on previously recorded dimensions (Dodd et al., 2009). For Loch Creran, well-formed reefs may reach heights of approximately 1 m, although they tend to fragment and collapse around 0.6 m tall (Moore et al., 2009). Thus, the experimentally measured value for area-specific serpulid carbonate mass was doubled, to produce the estimate of 6,931 t CaCO3 quoted above. If reef height for Loch Creran is assumed to be even higher (0.6-0.9 m tall), this would result in a potential standing stock of 1,247 t IC. Given the various uncertainties an order-of-magnitude estimate for standing stock of inorganic carbon in Scotland’s serpulid aggregations is therefore considered more appropriate of 1,000 t. A standardised IC density value can be crudely estimated of 11.5 t IC ha-1 for a 0.1 m tube height, using the mass-volume reported above; however, there is low confidence attributed to this value due to the irregularly shaped tube masses found within serpulid reef (Burrows et al., 2014).
Production of IC by serpulid aggregations in Loch Creran has been estimated at 420 g IC m-2 yr-1 (Burrows et al., 2014), the highest rate of all considered blue carbon habitats after kelp beds. This equates to a production capacity of 54.2 t IC yr-1 for the known Scottish extent. However, the production value used by Burrows et al., (2014) was based on another reef-building serpulid species, Galeolaria hystrix, and does not account for calcification or respiration. Therefore, large uncertainties remain. Evidence from Scotland and New Zealand suggests that serpulid skeletal carbonate can persist in sediments for several decades, but it is unlikely to be preserved for longer, as chemical dissolution and/or bioerosion ultimately leads to degradation (Burrows et al., 2014). Thus, this carbon may only be stored over a timescale of <100 years.
Threats to serpulid aggregations
Serpulid aggregations are considered a PMF in Scotland (SNH, 2018b), a Scottish Biodiversity List habitat, and as a protected feature within MPAs. Serpulid aggregations are highly sensitive to, and recover slowly from physical disturbance, such as from bottom-contact fishing activities (SNH, 2018b). Changes in water flow and salinity are also threats to this habitat. Pressures arising from the spread of INNS may also threaten serpulid aggregations. For instance, the invasive colonial sea squirt, Didemnum vexillum, has been recorded in Loch Creran (Begg et al., 2020). This has the potential to impact the rare serpulid reefs within the loch and a monitoring programme has been established to understand the potential spread (Moore & Harries, 2020).
Broader degradation of serpulid aggregations seen within Scotland may also be natural and part of a longer-term cycle (Moore et al., 2020). A case study is presented for Loch Creran within Scotland’s Marine Assessment, 2020 (SMA, 2020b). The action of storms on reef structures, perhaps exacerbated by the presence of colonising kelp plants, was suggested as a possible factor for declines observed in Loch Teacuis (Kamphausen et al., 2015).
A major threat to marine calcifiers is ocean acidification, with serpulids predicted to being highly vulnerable to changes in ocean chemistry predicted for the near future (Smith et al., 2013). Chemical dissolution of CaCO3 is expected to be most rapid in bioturbated terrigenous muds, typical of sea lochs (Aller, 1982). Ocean acidification will lead to a chemical environment, which favours dissolution, exacerbating the degradation of serpulid aggregations in Scottish sea lochs. Additionally, projected increases in storminess associated with climate change poses a threat to serpulids, resulting in more frequent collapse of aggregations and less time to rebuild (SNH, 2018b).
Future research on serpulid aggregations
Limited knowledge of Scottish serpulid habitat extent lowers the confidence associated with carbonate production estimates calculated. This is also made more challenging through the natural cyclical growth and collapse of reefs. Although serpulid aggregations of Loch Ailort may not cover as large an area as other Scottish sites, mapping and including this inorganic carbon standing stock is required to reduce uncertainties in estimates. Additionally, declines in both Loch Creran and Loch Teacuis have been reported (Moore & Harries, 2020; SNH, 2015; Tulbure, 2015). This should be investigated and assessed further, to improve the accuracy of values of total carbonate produced by Scottish serpulid aggregations. Serpulid aggregations have a restricted global distribution and are associated with a high biodiversity, which further supports the research needs.
As Scottish serpulid aggregations represent a relatively small amount of the total carbon stored within blue carbon habitats, perhaps less focus is needed on quantifying exact amounts. Instead, efforts should be directed towards reducing pressures and informing management decisions that protect these rare habitats. In relation to The Inshore Fishing – Prohibition of Fishing and Fishing Methods (Scotland) Order 2015, fisheries measures are already in place for Loch Creran and Loch Teacius. Discussions are ongoing around the introduction of fisheries measures in Loch Ailort.
4.5 Flame shell beds (Limaria hians)
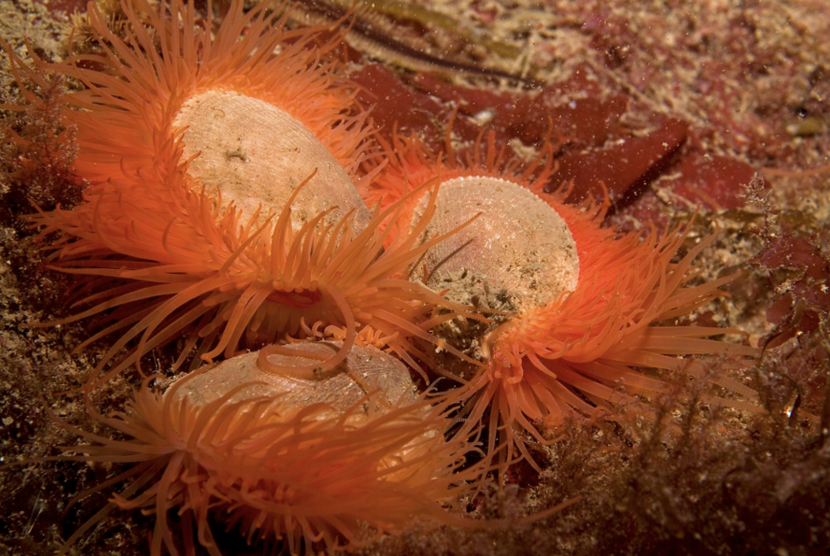
The flame shell Limaria hians is an epifaunal bivalve that constructs nests formed by the intertwining of byssal threads with seaweed, maerl, shells and stones. Large structures can form between adjoining nests if conditions allow, covering the seabed. These carpets create a habitat, which supports a high biodiversity by providing shelter and complex structures (SNH, 2018g).
Distribution and extent of flame shell
Historical records are widespread around the western and northern Scottish coasts (Hall-Spencer and Moore, 2000b). Scottish flame shell beds are believed to have suffered a severe decline in abundance, with few extensive sites known to exist (SNH, 2018g; Burrows et al., 2014). The Gutter Sound flame shell bed in Scapa Flow is the largest in Scotland, conservatively estimated to cover 508 ha (GeMS V8_i24). The total predicted extent of flame shell bed habitat across Orkney waters (90% probability of occurrence) is 1,799 ha (Porter et al., 2020). Several dense populations in west coast sea lochs have been located, with the second largest-known bed covering an estimated 194 ha within Strome Narrows and its western approach channel as part of the Loch Carron MPA (Moore et al., 2018). Other locations and extents of Limaria beds in Scotland have been previously summarised (Moore et al., 2013; Moore et al., 2020), with all known sites compiled in Table 2. The total extent of flame shell beds is estimated to be approximately 2,269 ha.
Table 2. Location and extent of known and in some cases predicted [c] Scottish flame shell beds (Burrows et al., 2014; Moore et al., 2013; Moore et al., 2018; Moore et al., 2020; Porter et al., 2020). The average carbon content as mass values per individual have been taken from Porter et al. (2020) to calculate the standing stock values (0.197 g IC and 0.716 g OC) by multiplying by total number of individuals. [a]represents area of habitat where >50% cover of nest material, [b]represents the area of habitat supporting >10% cover of nest material, [c] based on MaxEnt modelling and >90% probability of occurrence. Caution is advised when comparing values that are derived using different methodologies, particularly modelling results.
Location | Year | Bed Extent (m2) | L. hians density (ind. m-2) | Total number of L. hians | Standing stock IC (t) | Standing stock OC (t) |
---|---|---|---|---|---|---|
Loch Broom |
2010 |
~70,000 [a] |
97 |
6.79 x 106 |
1.34 |
4.86 |
Loch Alsh |
2012 |
930,000 [a] |
275 |
2.56 x 108 |
50.38 |
183.12 |
Laudale Narrows, Loch Sunart |
2006 |
200,000 [a] |
229 |
4.8 x 106 |
0.95 |
3.44 |
Port Appin, Loch Linnhe |
See below [c] |
~30,000 [a] [d] |
343 |
1.03 x 107 |
2.03 |
7.37 |
Shian and Creagan Loch Creran |
2019 |
350,000 [a] |
300 [e] |
1.05 x 108 |
20.69 |
75.18 |
Otter Spit, Loch Fyne |
2012 |
270,000 [a] |
367 |
9.91 x 107 |
19.52 |
70.95 |
Inner Sound, Ross and Cromarty |
2017 |
710,000 [b] |
300 [e] |
2.13 x 108 |
41.96 |
152.51 |
Strome Narrows, Loch Carron |
2017 |
1,940,000 [b] |
330 |
6.4 x 108 |
126.12 |
458.38 |
Sgeir Bhuidhe, Loch Carron |
2017 |
200,000 [b] |
420 |
8.4 x 107 |
16.55 |
60.14 |
Orkney |
2020 |
17,990,000 [c] |
412 |
7.4 x 109 |
1,460.16 |
5,306 |
Total |
– |
22,690,000 |
– |
– |
1,740 |
6,323 |
[d] Bed extent for Port Appin was measured in August 2011 (L. hians density not recorded). The value for L. hians density is the most recent measurement (February 2007).
[e] L. hians density not recorded, so average of all other densities from the table taken.
Blue carbon contributions from flame shell
At the time of the review by Burrows et al. (2014), no published data for shell weight of L. hians were available. Instead, standing stock carbonate calculations were based upon data for L. fragilis (a species of similar size to L. hians) and the typical carbonate percentage of mollusc shells. A value of 1.7 g was therefore used to represent the carbonate content of a L. hians shell, which equates to 0.2 g of IC (Burrows et al., 2014). Since that review, the Heriot-Watt Scientific Dive Team have collected L. hians samples from a location in Lyness (Porter et al., 2020). Average OC content of shell and tissue per individual was found to be 0.716 g, with the average IC content measured as 0.197 g (Porter et al., 2020). This latter value is comparable to the estimate from Burrows et al. (2014). Using the reported values for the organic and inorganic content of L. hians, a total carbon standing stock has been calculated by multiplying by the approximate number of individuals at each site (Table 2). Scottish flame shell beds store approximately 8,000 t of total carbon within soft tissue and shell, of which, ~6,323 t is organic and ~1,740 t is inorganic (Table 2). This may represent an overestimate, as differing values for bed coverage were used when assessing habitat extent. Burrows et al. (2014) gave values for extent where coverage >50%, whereas the values from Moore et al. (2018) are for coverage >10%. This may lead to over-estimations of the standing stocks calculated for their sites. Average cover was reported between 30-70% for these sites, with extensive areas supporting coverages of around 100% (Moore et al., 2018), so this may not represent a large inaccuracy. Additionally, the Inner Sound bed is among the larger beds known (Moore et al., 2018), however, low sampling intensity and no reported value for L. hians density, places high uncertainty on the standing stock estimate at this site.
The number of individuals per square metre varies between 31 and 420 for each site surveyed (Table 2) highlighting the large degree of uncertainty in calculating an average carbon density value for this habitat. From direct analyses, Porter et al. (2020) estimated an average carbon density of 0.61 t IC ha-1 and 3.3 t OC ha-1 for the Orkney flame shell population for a nest thickness of 0.21 m. (These values were presented the wrong way round in Porter et al., (2020) and have been corrected here (Jo Porter, 2022, personal communication)). Standardised to 0.1 m, this equates to approximately 0.3 t IC ha-1 and 1.57 t OC ha-1, representing the best available estimate for flame shells in Scotland currently.
The nests secreted by flame shells, made up of byssus thread and trapped shell, algae, and sediment contain a significant amount of carbon (Porter et al., 2020). No research has been undertaken to understand the fate of this carbon and as such, it has not been included within this review. The thin, delicate shells of L. hians are likely to persist for timescales of only years to decades. This coupled with the lack of published data on individual growth or settlement rates, results in an unknown sequestration rate (Burrows et al., 2014).
Threats to flame shell
Flame shell beds are considered to be marine conservation priority habitats; as a PMF in Scotland (SNH, 2018g), a Scottish Biodiversity List habitat (as file shell beds), and as a protected feature within MPAs. In one study Limaria nest regrowth over experimentally-cleared areas was found to be slow, averaging approximately 3.2 cm yr-1 (Trig and Moore, 2009). It was also noted that recruitment was low, with the bed expansion resulting from established flame shells spreading from undisturbed areas instead. However, recovery rates could be higher where healthy material is adjacent providing a possible mechanism for enhanced recruitment as proposed by Moore et al., (2018) following post-disturbance surveys in Loch Carron. The slow rate of regrowth highlights the vulnerability of this habitat to physical disturbance, with dredging leading to high levels of mortality, which is believed to be the main contributing factor in flame shell decline in the UK (Hall-Spencer and Moore, 2000b). Flame shell beds are also highly sensitive to siltation, smothering, wave action and changes in water flow (FeAST, 2013; Mazik et al., 2015).
As Limaria is not expected to persist long after death, flame shell beds may act as a modest carbon store, only when occupied by dense and healthy populations. Destruction or severe disturbance may lead to the remobilisation of this stored carbon (Burrows et al., 2014).
Future research on flame shell
There are uncertainties in calculating total standing stock of carbon within Scottish flame shell beds, arising from differing Limaria coverage and the possibility of unrecorded sites. It is also not known what the fate of the carbon stored within the living tissue is after death. Although no sequestration rate has been calculated in this review due to a lack of published data on growth or recruitment, the slow regrowth of this species highlights a more pressing matter. As L. hians is unlikely to persist long after death, leading to the remobilisation of previously stored carbon, it is more important to protect the existing beds and prevent any pressures that result in physical disturbance.
4.6 Horse mussel beds (Modiolus modiolus)
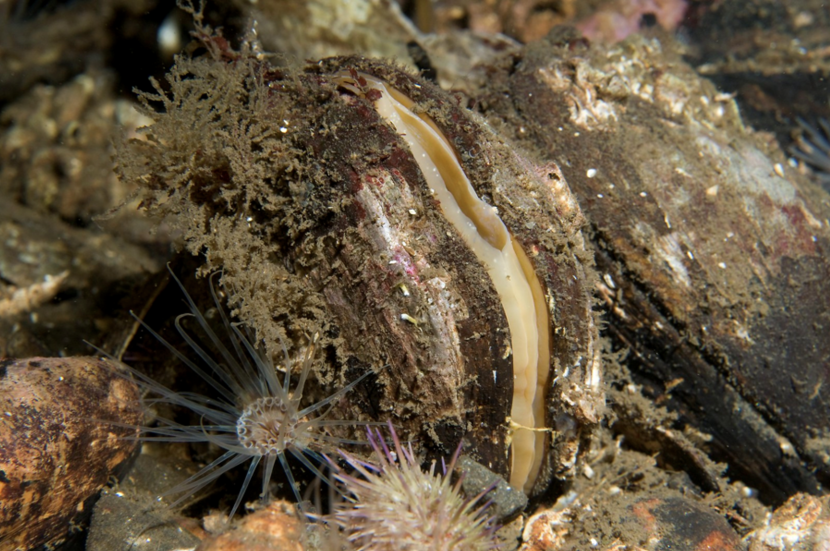
Horse mussels fund together by a matrix of byssus threads to form raised beds. These can form scattered clumps, think layers or dense raised beds, which accumulate in height through the deposition of silt, pseudo-faeces and shells within the bed (SNH, 2018f).
Distribution and extent of horse mussel beds
The horse mussel Modiolus modiolus is widely distributed in shallow subtidal environments around Scotland, particularly along the northern and western coasts, including Orkney and Shetland (Mair et al., 2000; SNH, 2018f). Many records are of isolated individuals or sparse low-density patches, but beds dense enough to be regarded as calcifying aggregations are present in some locations (Burrows et al., 2014). A recent study combined the extent of known sites (e.g. North Cava Island bed of 71 ha - GeMS V8_i24) and predictive modelling results to estimate 3,828 ha (probability of occurrence > 0.9) of horse mussel bed coverage within Orkney waters (Porter et al., 2020). Although a limited number of the predicted areas have been ground-truthed by drop down video surveys, in situ diver video, or photographs, the extent is still likely to be an underestimate due to a complete absence of predicted modelling for water depths greater than 50 m (Porter et al., 2020). However it is also prudent to note that there is likely an element of double-counting where multiple habitats co-exist; for instance within Gutter Sound, horse mussel beds and intermixed with flame shell beds, neither of which therefore have 100% coverage. This is a limitation of MaxEnt modelling, that predictions on the likelihood of suitable habitat may be the same for multiple habitat types, without inferring which habitat may be more likely. The horse mussel bed off Noss Head, Caithness, is the next largest currently known in Scottish waters, with an estimated extent of approximately 410 ha (SNH, 2018f). The combined extent of 4,238 ha may be a conservative estimate of extent given other known but much smaller beds have not been included. However, the issue of double-counting mosaic habitat must also be considered.
Blue carbon contributions from horse mussel beds
Density of living horse mussels at Noss Head is patchy, but the SACFOR category of Superabundant (10-90 individuals m-2) was recorded at numerous sites along the bed (Hirst et al., 2012). The M. modiolus bed off Pen Llŷn, North Wales, covers about the same area as Noss Head, with a characteristic undulating bedform of ridges and troughs, which are features present at Noss Head also (Lindenbaum et al., 2008; Hirst et al., 2012). At Pen Llŷn, shelly reef deposits are 0.5-1 m deep depending on the surface undulations (Lindenbaum et al., 2008), with a mean depth assumed to be 0.75 m. Based on ~0.05 m deep grab samples in the Firth of Lorn, M. modiolus has an area-specific density of 2,219 g CaCO3 m-2 or 266 g IC m-2 (Collins, 1986). If Noss Head is assumed to have a similar mean depth as Pen Llŷn of 0.75 m, this equates to a standing stock estimate of 4,000 g IC m-2 (equivalent to 40 t IC ha-1) for the entire depth (Burrows et al., 2014). However, this estimate is likely at the upper limit as horse mussel density is not uniform across bed extent. Standardised to 10 cm, we estimate an approximate density of 5.33 t IC ha-1, which is based on several assumptions detailed above, and therefore has low associated confidence. Across the estimated extent of habitat, 4,238 ha, and assumed mean bed thickness of 0.75 m, this review estimates a total standing stock of 169,520 t IC within Scottish horse mussel beds.
Due to a lack of available data within the literature for the organic carbon component of horse mussel tissue, the recent blue carbon audit for Orkney attempted to fill this knowledge gap (Porter et al., 2020). Utilising LOI burn ups on 6 individuals collected near Cava Island and assessing the literature for the relative composition of C:N:P in the tissue of the closely related genus Mytilus, the average organic carbon content within the tissue of an individual horse mussel is approximately 6.3 g ± 3 g OC. For the Orkney horse mussel population, a standing stock of 348.63 g OC m-2 was estimated (Porter et al., 2020); although it is not clear what thickness of bed this estimate is derived for.
Horse mussels are long-lived and relatively slow-growing, resulting in a low area-specific carbonate production rate, estimated as 330 g CaCO3 m-2 yr-1 in the Firth of Lorn (Collins, 1986). This equates to an inorganic carbon production rate of approximately 40 g IC m-2 yr- 1, nottaking into account CO2 released during calcification. The total carbon production capacity for the reported extent of 4,238 ha is therefore 1,695 t IC yr-1. Thick deposits of M. modiolus are unlikely to persist for long enough to enter the geological record, but do have the potential to store inorganic carbon over a timescale of ~1000 years (Burrows et al., 2014).
Threats to horse mussel beds
Horse mussel beds are considered to be marine conservation priority habitats; as an OSPAR threatened and/or declining habitat, PMF in Scotland (SNH, 2018f), a Scottish Biodiversity List habitat, and as a protected feature within MPAs.
Distribution of horse mussel beds is thought to be correlated with water temperature (Brown, 1984), indicating a potential vulnerability as sea temperature increases with climate change. Under projected climate change scenarios, modelling predicted that suitable habitat for horse mussel beds in the UK would be entirely lost by 2080 (Gormley et al., 2013). Ocean acidification is another climatic-driven pressure, with significant changes found in shell morphology and thickness of the blue mussel Mytilus edulis under reduced pH (Fitzer et al., 2015). This could increase vulnerability to predation, and pH would likely have a similar impact on M. modiolus. Other climatic pressures arise from increased occurrence of hypoxic events and changing ocean currents (FeAST, 2013; Wildish et al., 2000). Horse mussel beds are also sensitive to physical disturbance and siltation changes, with aquarium experiments finding over 50% mortality of M. modiolus after 16 days of burial (Hutchinson et al., 2016). The presence of some INNS, such as D. japonica and C. eumyota, may result in additional pressures for horse mussels (and shellfish more generally) in Scotland through competition for food and space (Collin et al., 2015).
Future research on horse mussel beds
Research should be focused on fully mapping the known locations of horse mussel beds, with increased effort into surveying other suitable areas for beds also. A study looking at the genetic connectivity between horse mussel beds highlighted connections between horse mussel beds within MPAs and nearby populations outside the protected areas (Mackenzie et al., 2018), providing useful insights into species recruitment. Such information can help to inform future survey effort. Improved extent mapping will inform the total carbon standing stock of this habitat. Furthermore, the impacts of climate change and multiple stressors on horse mussel beds should be investigated, to understand what degree of change they can tolerate and how their distribution may change in the future.
4.7 Blue mussel beds (Mytilus edulis)
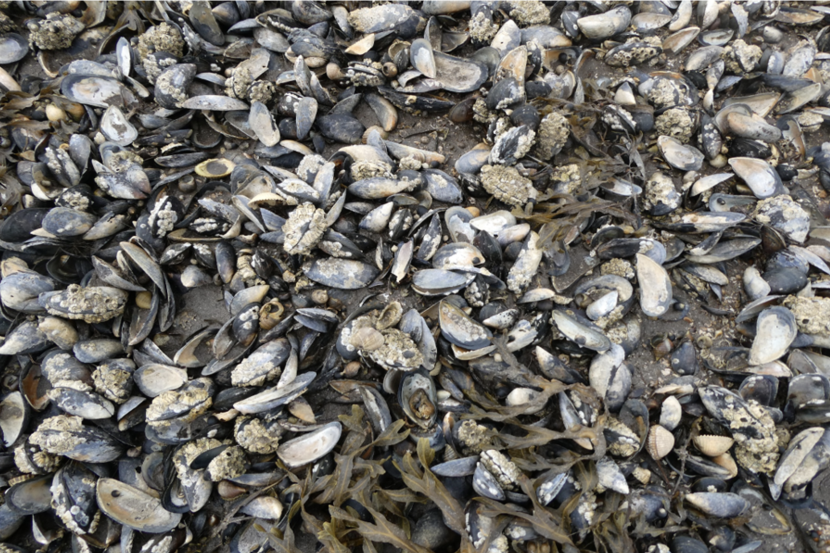
Individual mussels can form beds or reefs in the intertidal zone composed of a single or multi-layers, which are held together by byssus threads. The bed stabilises sediments and creates a habitat with high diversity living on, within or under the bed (SNH, 2018c).
Distribution and extent of blue mussel beds
The blue mussel Mytilus edulis can form beds on intertidal or subtidal sediments when in high densities, using byssal threads to form attachments. This creates a distinctive matrix that stabilises sediment and accumulates organic detritus and shell debris (SNH, 2018c). In Scotland, blue mussel beds are found in scattered locations along the coast, particularly at the head of sea lochs and in the mouths of estuaries and firths. Focusing on subtidal beds, the largest known (generated through broadscale mapping) is the Firth of Tay at >400 ha, with other discrete beds exceeding 35 ha formerly present in the Dornoch Firth (SNH, 2018c). For Nigg Bay, Cromarty Firth, densities of M. edulis vary from 100 to 1000 mussels m-2 (Trendall et al., 2011). On the west coast, notable sites include the Solway Firth and several sea lochs (McKay and Fowler, 1997), and populations have been recorded around Shetland, Orkney and the Outer and Inner Hebrides. There is a significant lack of national extent mapping for this habitat, as well as observed trends of habitat decline from recent survey work (Burrows et al., 2017), thus assessments of the carbon standing stock are not currently possible.
Blue carbon contributions from blue mussel beds
There is currently very limited research on the carbon cycling of Mytilus edulis beds and therefore the blue carbon contribution of this habitat is unknown. This currently represents a significant knowledge gap. A literature review carried out for Dutch cultivated and wild mussels revealed the complexity of metabolic processes as well as the physical environmental conditions that need to be accounted for to understand the net carbon status of blue mussels (Jansen & van den Bogaart, 2020). Carbon sequestration values varied for instance depending on whether shell formation only, or metabolic processes such as respiration, tissue production, bio-calcification and/or bio-deposition of (pseudo)faeces were included in the model, and even whether the mussels were in a sheltered or exposed location. Mussel shell morphology could also be affected by cultivation method, as it was noted that bottom cultivation resulted in thicker shells and therefore more stored IC, compared to rope-grown mussels, which grow faster but have thinner shells (Jansen & van den Bogaart, 2020). Depending on the accounting approach used, the carbon capture potential for an individual Dutch mussel over two years varies between -540 and 1,030 mg carbon, affecting whether it is considered a carbon source or sink. The values reported from the literature review for inorganic carbon sequestration via carbonate shell formation only (i.e., reported as carbon assimilation in shell material (gross)) varied between 3.8 - 129.2 g IC m-2 yr-1 depending on location exposure for one study (Hily et al., 2013). Jansen & van den Bogaart’s review (2020) reported an average inorganic carbon assimilation of 62.9 g IC m-2 yr-1. Other assessments have opted to make assumptions based on horse mussel sequestration rates instead, taking 10% of the horse mussel value on the basis that they are significantly larger than blue mussels (Armstrong et al., 2020).
The value of 62.9 g IC m-2 yr-1 will be taken to represent a mean IC production rate for blue mussels in this review, although it is noted that this is based on a Dutch population and does not account for any CO2 emissions during calcification or metabolic processes. Hence, confidence is low.
Threats to blue mussel beds
Blue mussel beds are a PMF in Scotland (SNH, 2018c) and recognised as a protected feature within 38 MPAs. They are also an OSPAR threatened and declining habitat (littoral beds on mixed or sandy sediment). Despite this afforded protection, blue mussel beds are declining in Scotland (Burrows et al., 2017) following a similar trend that has been seen more widely for this species throughout the North Atlantic area (Baden, Hernroth & Lindahl, 2021). Further work is being led by NatureScot to determine the causes of declines within Scotland, which are currently indeterminate. Initial investigations will try to understand the risks to the species from the likes of climate change, pollution, predation, over-fishing, invasive species, disease, and recruitment.
This habitat is sensitive to various pressures, including to marine INNS such as the slipper limpet Crepidula fornicata, which has the potential to out-compete blue mussels (Mainwaring et al., 2014), but has not yet been recorded in Scotland. Given its established presence in other regions of the UK (Mainwaring et al., 2014), the slipper limpet is a relevant threat with potentially high impacts. Other INNS also pose a potential risk to blue mussels (Collin et al., 2015). Blue mussel beds may also be vulnerable to climatic-driven pressures including sea level rise, increasing water temperatures and ocean acidification (SNH, 2018c). Significant changes in the shell morphology and thickness of blue mussels were observed under a reduced pH (Fitzer et al., 2015). This could leave the mussels more vulnerable to predation and more likely to fracture in stormy conditions (Fitzer et al., 2015). Ocean acidification has also been shown to decrease mussel byssal attachment strength in the related species Mytilus coruscus (Zhao et al., 2017). This could increase the risk of dislodgment and hamper the formation of mussel beds. Other anthropogenic pressures such as towed bottom-contact fishing and anchoring could further impact blue mussel beds (SNH, 2018c).
Future research on blue mussel beds
Data are deficient for blue mussel beds and future research should focus on (re-)mapping the known sites within Scotland to produce an accurate extent estimate. A current PhD study ‘The Blue Carbon of Shellfish Beds: Understanding the vaults of biogenic reefs (O. edulis, M. modiolus and M. edulis)’ hosted by Heriot Watt University is expected to inform our understanding of the extent of beds in multiple east coast locations, as well as building on knowledge of carbon cycling within these marine bivalves. Furthermore, there is a clear lack of evidence regarding net sequestration rates or carbonate content for this species. Without this key information, deciding on the level of prioritisation over other blue carbon habitats is challenging.
4.8 Native oyster (Ostrea edulis)
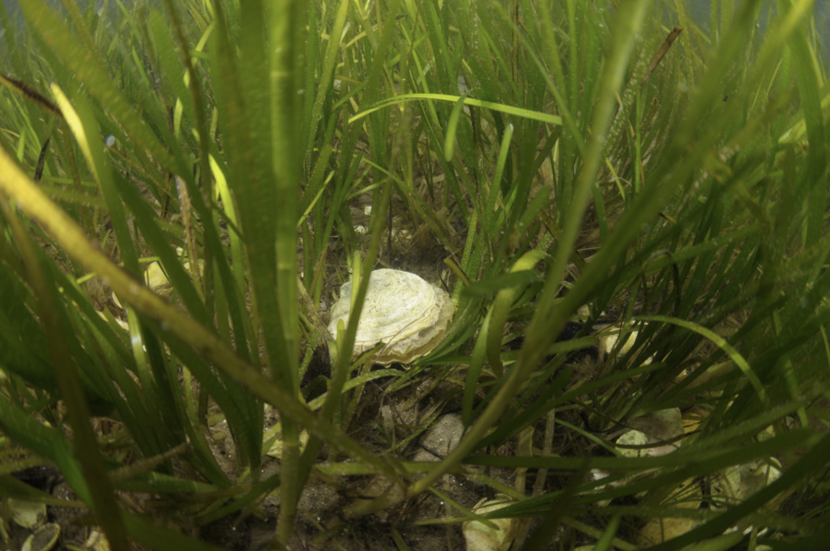
The native oyster, or European flat oyster Ostrea edulis, is a bivalve found in highly productive, shallow, estuarine and coastal waters (SNH, 2018d). Where conditions allow, dense beds may form, providing benefits including as nursery habitats, improving water quality and contributing to carbon sequestration (zu Ermgassen et al., 2020).
Distribution and extent of native oyster
Within Scotland, the native oyster range is significantly reduced compared to historical accounts (Fariñas-Franco et al., 2018). This is a result of overfishing during the late 1800s and early 1900s, along with pollution, disease and pests (SNH, 2018d). Beds are currently constrained to the west coast and islands (University Marine Biological Station Millport, 2007), with records in Loch Ryan, Loch Sween, Loch Scridain and Loch Eishort (SNH, 2018d).
Scottish beds may be large (up to 15 km2, 1500 ha) or occur as small, fragmented groups around the edges of sheltered lochs (SNH, 2018d). Loch Ryan is an actively managed fishery and hosts the largest extant native oyster bed in Scotland. The Lefnoll area alone is estimated to cover an area of 1.6 km2 (160 ha), with densities of 3 adults and 30 spat m-2 (University Marine Biological Station Millport, 2007). Extensive mapping of this habitat is lacking for Scotland, thus assessments of the carbon standing stock are not currently possible.
Blue carbon contributions from native oyster
Research pertaining to carbon content values and sequestration rates of the native oyster is limited. Estimates of carbon stock and carbonate production rates are therefore not available. However, a recent ex situ study found that live native oysters were responsible for tripling sediment deposition from the water column, facilitating the transport of OC and IC to the seabed. The experiment occurred over ten days, with a continuous flow-through of seawater to deliver food particles. Live, dead, and control treatments were used to account for background deposition. Three oysters were deployed for the live and dead groupings, scaling to a density of 75 oysters m-2. Quantification of carbon within the sediment was carried out using CHN elemental analysis (Lee et al., 2020). A total carbon deposition rate of 50 g C m-2 yr-1 for the native oyster was calculated from the experiment, which included both organic (~65%) and inorganic (~35%) forms (Lee et al., 2020). Given the highly scaled density, this value for carbon sequestration is likely not representative of current Scottish oyster beds, however does indicate that native oysters play a supporting blue carbon role in facilitating sediment draw down and the potential burial of organic carbon, although labile ‘fresh’ carbon is readily remineralised. In a natural oyster bed, further variability in benthopelagic coupling may arise through differences in food availability, seasonality and community processes such as bioturbation (Lee et al., 2020).
Threats to native oyster beds
Both as a species and a habitat, native oysters are recognised as threatened and/or declining under OSPAR, a PMF and a feature within the Loch Sween MPA. Native oyster beds are considered under threat in all regions where they occur (SNH, 2018d). Unlawful gathering of oysters on a wide scale poses a threat to populations, with evidence of this illegal activity occurring in Scotland (Lancaster et al., 2014). Physical disturbance through bottom-contact fishing has the potential for significant impacts on native oysters (SNH, 2018d). However, given the limited distribution of native oyster beds, exposure to bottom-contact fishing practises in Scottish waters is likely to be relatively low (SNH, 2018d).
Additional threats to native oyster populations in Scotland come from marine INNS, such as the slipper limpet or to less of an extent, the American whelk tingle (Mazik et al., 2015). Introduced pathogens (e.g. Bonamia ostreae) also pose a significant threat to population recovery (Mazik et al., 2015). It seems unlikely that parasites from the genus Bonamia will be strongly negatively impacted by climate change in the near to medium term (Arzul et al., 2009). However, research on the interactions between the parasite and native oysters under various climatic change scenarios is lacking.
The species is also sensitive to local water flow, temperature, siltation rate, pollution, and organic enrichment (Mazik et al., 2015; SNH, 2018d). Climatic pressures including changes to sea level, water temperatures, ocean acidification and increased storminess, again pose threats to native oysters (Lemasson et al., 2017; SNH, 2018d).
Restoration of native oyster
The diminished status of native oyster beds has resulted in the recent popularity of restoration projects, including the NORA consortium in Europe (Pogoda et al., 2019), and the Dornoch Environmental Enhancement Project (DEEP) in Scotland (Fariñas‐Franco et al., 2018). A further marine restoration programme in the Firth of Forth, Restoration Forth, is underway and aims to restore 30,000 native oysters by 2024. Factors influencing the recovery of the native oyster include: brood stock size; availability of preferred settlement surfaces; larval retention, and adult mortality (Mazik et al., 2015; Rodriguez-Perez et al., 2019; 2020). These factors and associated bottlenecks of restoration project licensing must be considered during any reintroduction project. Further restoration project considerations are presented within NatureScot’s Marine and Coastal Enhancement Project Assessment Framework (Chambers et al., 2022b)
As mentioned previously, the parasite Bonamia ostreae causes severe disease to native oysters and is prevalent in European waters (Mazik et al., 2015; Sas et al., 2020). Current oyster restoration projects aim to increase density to a self-sustaining population level. Since parasite prevalence likely increases with density (Engelsma et al., 2010), the risk of disease amongst restoration projects may also increase (Sas et al., 2020). Restoration projects should comprehensively consider the risk posed by B. ostreae and avoid further spreading (Sas et al., 2020).
Future research on native oyster
Understanding of the blue carbon service provided by native oyster beds is currently limited. A current PhD study ‘The Blue Carbon of Shellfish Beds: Understanding the vaults of biogenic reefs (O. edulis, M. modiolus and M. edulis)’ hosted by Heriot Watt University is expected to improve our understanding of carbon budgets within these marine bivalves. Further work is needed to assess how depositional rates may change seasonally and alongside other environmental variables. Given the recent escalation of native oyster restoration projects, research should aim to quantify the ecosystem services associated with these beds and integrate these estimates into marine policy. A study of this nature has recently demonstrated the biodiversity net gain that occurs as a result of native oyster reef development within a long-established dredge fishery (Kennon et al., 2023). Biodiversity was shown to increase with time since harvest and predicted to double within 10 years, attributed to the provision of structural complexity from live and dead shells. This research will help to inform sustainable fishery management towards environmental health, as well as supporting restoration for biodiversity benefits. Finally, future work should also investigate the synergistic pressures facing native oysters, including how climate change may influence the parasitic spread of Bonamia.
4.9 Brittlestar beds (Ophiothrix fragilis)
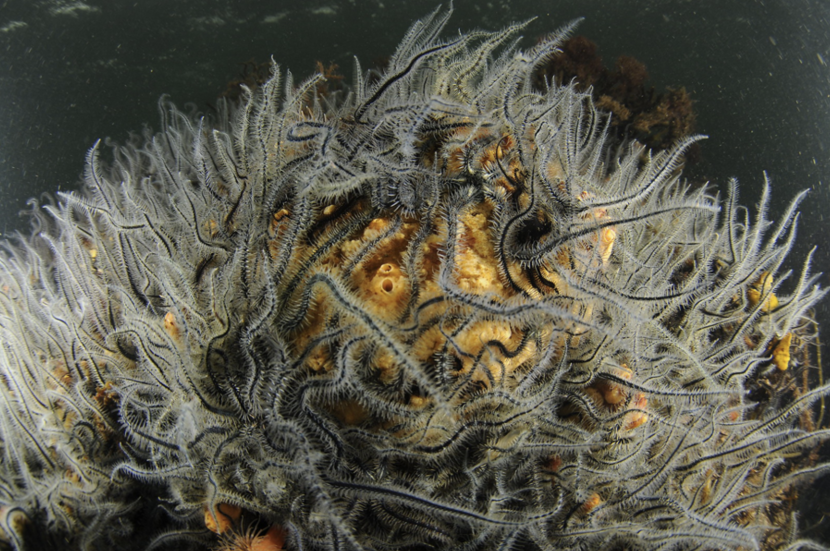
Although brittlestar beds are not strictly biogenic reefs defined by Holt et al. (1998), they do represent substantial concentrations of benthic biomass that may play a role in marine carbon cycling (Lebrato et al., 2010).
Distribution and extent of brittlestar beds
Dense beds of brittlestars, mainly Ophiothrix fragilis and Ophiocomina nigra, are common in Scottish waters along the west coast, and also in some areas of Orkney, Shetland and the North Sea (Hughes, 1998). Scottish spatial extent of brittlestar beds is largely unknown. However, values have been recorded elsewhere in the UK. In the Dover Strait and eastern English Channel, O. fragilis populations have been estimated to cover 60,000 ha and 540,000 ha respectively (Sanvicente Anorve, 1995; cited in Migné et al., 1998).
A recent study by Porter et al. (2020) assessed the blue carbon contribution within Orkney waters. This study provides the only supporting data for both brittlestar beds and the subsequent section on Bryozoa. In both cases, the distribution of key species was predicted using MaxEnt species distribution modelling. In Orkney waters, 4,756 ha distribution of O. fragilis was predicted using MaxEnt species distribution modelling (Porter et al., 2020).
Blue carbon contributions from brittlestar beds
A survey of a bed north of Cava Island conducted in 2019 by the Heriot Watt Scientific Dive team, recorded a density of 240 ind. m-2 for O. fragilis (Porter et al., 2020). Brittlestar bed densities in other parts of the UK have been recorded as significantly higher. For instance, surveys of O. nigra off Keppel Pier, Great Cumbrae, were recorded exceeding 2000 ind. m-2 (Aronson, 1989) and a bed of O. fragilis in the Dover Strait averaged 1,229 ind. m-2 (Migné et al., 1998). This latter value represents a carbonate density of 555 g CaCO3 m-2, which is equivalent to 66.2 g IC m-2 or 0.66 t IC ha-1 (Burrows et al., 2014).
More recently, a study applying LOI burn up experiments to O. fragilis sampled from Cava Island, Orkney waters found an average total carbon value of 0.57 g per individual comprising ~ 42% OC and ~ 58% IC. Multiplying this by an estimated 240 individuals per m2 gives an overall carbon density, of about 135 g C m-2 (Porter et al., 2020); this represents an IC density of approximately 79.19 g IC m-2, or 0.79 t IC ha-1. The sample size of this was low (n=5), however there is good agreement with the IC density of the Dover Strait (Migné et al., 1998). Porter et al. (2020) estimate a total carbon standing stock of 6,460 t for the predicted extent of brittlestar beds within Orkney waters. This estimate is based on predictive distribution modelling and only accounts for Orkney waters, but this highlights that the potential contribution of brittlestar beds to carbon storage in Scottish waters should warrant further consideration.
Migné et al. (1998) estimated a value of 682 g CaCO3 m-2 yr-1 for the Dover Strait O. fragilis bed. This equates to a carbon sequestration rate of 81.84 g IC m-2 yr-1 (Burrows et al., 2014). Brittlestar fragments will be subject to both bioerosion and chemical dissolution, with the longevity of their persistence in sediments depending on local environmental factors and the potential for burial below the Taphonomically Active Zone (Walker and Goldstein, 1999).
Threats to brittlestar beds
Brittlestar beds are not recognised as PMFs within Scotland and therefore are not targeted for protection from relevant marine activities. Incidental protection is likely afforded where brittestars co-exist with other features of conservation interest, particularly within MPAs e.g. where overlying flame shell beds, horse mussel beds or rocky reefs. As with all calcifying aggregation forming species, ocean acidification poses a threat to brittlestar beds. Exposure to a lower pH resulted in larval mortality, temporal decrease in larval size, and abnormal development of O. fragilis (Dupont et al., 2008). A review by De-Bastos and Hill (2016) assessed the various sensitivities of the bed-forming species O. fragilis and O. nigra. It was noted that passing scallop dredges are likely to remove, displace, or damage brittlestars (De-Bastos and Hill, 2016). The relatively sessile O. fragilis was shown to decrease over the long-term in areas subject to dredging (Bradshaw et al., 2002). Increased sedimentation may also lead to smothering of brittlestar beds, with Aronson (1989) associating the decline of an Ophiothrix bed in Torbay with increased sedimentation from the localised dumping of construction materials.
Future research on brittlestar beds
Efforts should focus on mapping known brittlestar beds within Scottish waters, as little is available about extent. Revisiting previous records would also inform understanding of temporal persistence (Hughes, 1998). Sequestration rates for both bed-forming species at sites in Scotland should also be calculated, to improve estimates. Although Porter et al. (2020) calculated the carbon content for brittlestars in Orkney, the LOI experiments only used five individuals. A higher sample size should be sought in future studies. As so little is known about brittlestar beds from a blue carbon perspective, including longevity and fate of carbon, difficulties arise when assessing the level of priority compared to other blue carbon habitats.
4.10 Bryozoan thickets (Flustra foliacea)
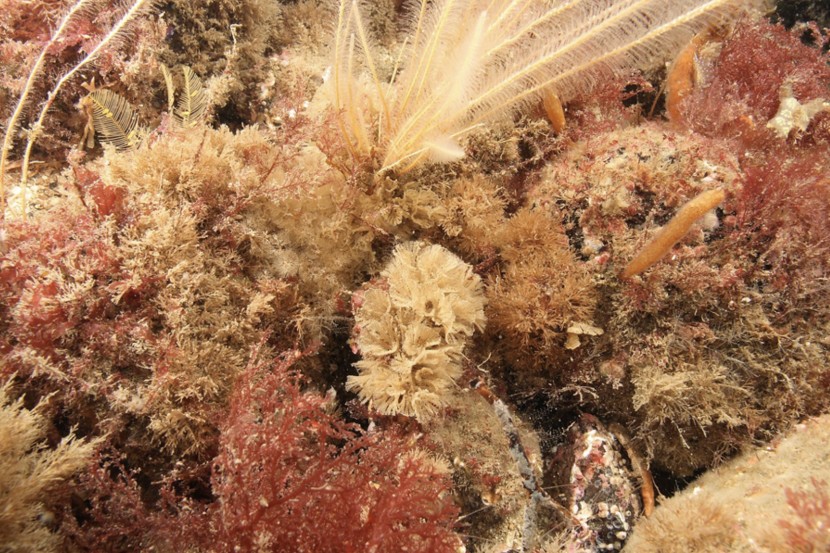
Bryozoan communities can form various types of thickets or turfs. Within Scotland, the first type is dominated by large erect colonies attached to bedrock or large boulders, including species such as Hornwrack Flustra foliacea and the closely related Securiflustra securifrons (Porter et al., 2020). The skeleton of Flustra foliacea is composed of calcium carbonate and chitonous material giving a flex that allows survival in strong tidal regimes. Another type of bryozoan thicket forms relatively short turf, often found as an understorey in kelp forests or as coatings on the surfaces of deeper rocky substrates (Porter et al., 2020). This type of turf consists of a variety of species mixes, with Bugulina flabellata, Scrupocellaria reptans, Crisia spp., Bicellariella ciliata and Cellaria spp. frequently present (Porter et al., 2020). A third type of thicket occurs mainly along the west coast of Scotland, dominated by bryozoan species such as Pentapora foliacea or Omalesecosa ramulosa, which form twiggy branched or coral-like colony structures (Porter et al., 2020).
Distribution and extent of Bryozoan thickets
Bryozoans have been recorded along all Scottish coasts and archipelagos, with the majority of records from the west coast and in Shetland, Orkney and the Western Isles (Rouse et al., 2014). They are suspension feeders and are adapted to surviving in strong tidal conditions, often attached to suitable hard substrata (Wood et al., 2012). Using a MaxEnt predictive model, the overall coverage of F. foliacea in Orkney waters was estimated as 9,416 ha (Porter et al., 2020). However, estimates of the full Scottish extent are unknown, so a carbon standing stock cannot be calculated.
Blue carbon contributions from Bryozoan thickets
Performing LOI analysis on subsamples taken from the dense turf of F. foliacea colonies in Orkney, gave an estimated area-specific density of 503 g C m-2, including both OC (473 g m-2 / ~94%) and IC (30 g m-2 / ~6%) components (Porter et al., 2020). The inorganic carbon fraction only makes up a small fraction of the species total carbon with Porter et al. (2020) reporting an IC density of 0.3 t IC ha-1 compared to 6.6 t OC ha-1.The sample size of this is unknown, as well as the average thickness, so a low confidence attributed to this estimate. However, Porter et al. (2020) notes that it is likely to be an underestimate. If this is applied to the predictive extent of F. foliacea coverage in Orkney waters, a total carbon standing stock of 47,362 t can be estimated for this region (Porter et al., 2020).
Threats to Bryozoan thickets
Bryozoan thickets are not recognised as PMFs within Scotland and therefore are not afforded direct protection from relevant marine activities. Protection is however afforded indirectly to bryozoan thickets as typical species of other features of conservation interest, particularly within MPAs (e.g. Annex I reefs). The majority of bryozoans are entirely calcitic, with the remainder either entirely aragonitic or bimineralic (Smith et al., 2006). Differing mineral compositions might be expected to affect dissolution rates of bryozoan carbonates; however, laboratory experiments have shown that the reactive surface area determines the dissolution rate more than mineralogy (Smith and Garden, 2013). In general, decreasing pH causes corrosion, changes in mineralogy, and reduced survival of bryozoans (Smith, 2014). This highlights the need for further research to better discern the risk of ocean acidification to Scottish bryozoan communities. Like with other epifaunal communities, physical disturbance by fishing gear has an impact, with bryozoan matrices greatly reduced in fished areas (Jennings and Kaiser, 1998). Additional threats identified within a global review highlighted excess sedimentation as a potential smothering mechanism of these suspension feeders, destruction during storms, salinity changes, and pollution, particularly from sewage (Wood et al., 2012). Bryozoans support diverse associated assemblages (Wood et al., 2012). Without a good understanding of their extent, a lack of targeted protection could have consequences for wider diversity and ecosystem service provision.
Future research on Bryozoan thickets
Clear evidence gaps have been highlighted after reviewing current literature. There is a lack of data on the current total extent of bryozoan thickets within Scottish waters. However, completing this mapping is acknowledged to be a challenging task. Work to improve understanding of the different types of thickets present in Scotland, as described by Porter et al. (2020), and their habitat requirements may help to inform future survey work and predictive habitat models. Sequestration rates have not been presented in this review due to data deficiencies in the literature. Therefore, efforts to calculate production and sequestration rates for the range of bryozoan species that characterise Scottish habitats is required. Work to classify the bryozoan species named with the three “types” (Porter et al., 2020) into biotopes to form features of the MPA network should be considered. This may help to support efforts for increased data collection, currently limited due to the lack of conservation designation status given to Bryozoa.
The estimated carbon standing stock of 47,362 t calculated for Orkney waters (Porter et al., 2020), is substantially larger than the carbon stored in most other Scottish calcifying aggregation habitats. The figure for bryozoan turfs represents only those predicted in Orkney waters, so the carbon contribution is likely much greater when taking into account other Scottish regions, such as the west coast. Furthermore, it likely represents an underestimate as only one species (F. foliacea) was considered. This highlights the potential importance of Bryozoa to Scottish blue carbon assessments, thus further research should be directed towards this habitat in this context.
5. Sediments
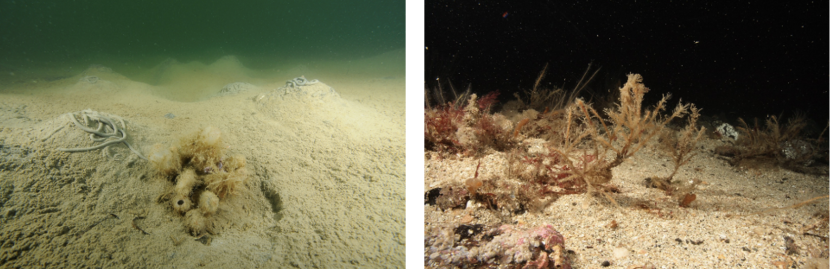
Sediments are considered separately to the blue carbon habitats previously discussed because they do not directly sequester CO2 from the atmosphere. Marine sediments are known to trap and store large quantities of carbon over long timescales >1000 years (Hedges et al., 1997). This buried carbon is derived from marine (Santschi et al., 1990; Glud, 2008; Krause-Jensen and Duarte, 2016), terrestrial (Bianchi, 2011; Bauer et al., 2013; Cui et al., 2016; Smeaton and Austin, 2017) and geological sources (Galy et al., 2015). Sediment type varies on the seabed as a function of surrounding hydrodynamic conditions, geological conditions, and underlying seabed morphology. Sediments are generally classified by the Folk sediment Classification depending on the relative contributions of gravel, sand, mud-sized grains (Kaskela et al., 2019).
Blue carbon contributions of sediments
The earliest attempt to map sedimentary carbon stores within Scottish territorial waters provided an estimate of 592 Mt OC and 1,739 Mt IC within the surficial sediments (top 0.1 m) (Burrows et al., 2014). This was based on a total area of approximately 470,000 km2, utilising substrate data derived from the British Geological Survey’s (BGS) database and considerations for unmapped offshore areas (Burrows et al., 2014). A further mapping study derived an improved estimate of 221 ± 92 Mt OC and 1,294 ± 161 Mt IC within surficial sediments of Scotland’s mapped extended Exclusive Economic Zone (EEZ) (Smeaton et al., 2020). This estimate was based upon an area of approximately 555,000 km2, which combined mapped data from BGS and data from the European Marine Observation and Data Network (EMODnet) for the EEZ extension (Smeaton et al., 2020). However, it did not include 40,180 km2 of unmapped Scottish seabed. A limitation of the study was the use of dry bulk density and %OC values taken from the eastern and southern North Sea compiled by Diesing et al. (2017) and did not include representative sediment types, such as those found in Scottish fjords. Adopting these values across the different sediment types and assuming a uniform OC content for the entire study area was reported to generate an underestimate.
Smeaton et al. (2021a) subsequently tried to reflect the various environmental differences leading to carbon variability in sediments by separating the seabed into three zones – fjords (covering Scottish sea lochs), coastal and inshore, and the wider shelf. Surficial sediment samples were analysed from across the entire Scottish sector of the UK EEZ (~458,000 km2) including almost 400 grab samples obtained from 18 surveys. This has been an underrepresented area in previous national sedimentary carbon stock estimates (Diesing et al., 2017). Smeaton et al., (2021a) used a spatial modelling approach to account for variability in sediment type and proximity to land. They found a mean value for surficial sedimentary stocks in Scottish adjacent waters of 356.5 ± 72.2 Mt OC (Smeaton et al., 2021a). These estimates are higher than those reported in Smeaton et al. (2020), likely due to the use of more site-specific OC and bulk density values from OC-rich coastal and muddy sediments around Scotland. The spatial modelling approach also provided an estimate for surficial IC stocks in Scottish adjacent waters of 2,264.8 ± 156.3 Mt IC (Smeaton et al., 2021a). This study supports the most comprehensive sedimentary carbon stock estimate to date for Scotland, and the wider UK EEZ.
Scottish fjords were included in the study by Smeaton et al. (2021a), comprising an area of 2,608 km2. Despite this relatively small area, they represent a stock of 3.9 ± 0.6 Mt OC and 5.3 ± 0.7 Mt IC in the surficial (0.1 m) sediment. This is comparable to the fjordic sediment stocks, 4.2 ± 0.5 Mt OC, of Smeaton and Austin (2019), so a high level of confidence can be placed in these values. When normalised for area, Scottish fjords hold 2,027 ± 367 t OC km-2, which is significantly higher than the value for the continental shelf, highlighting fjord sediments as hotspots for storing OC (Smeaton and Austin, 2019). Carbon stocks have further been estimated for the full depth of fjordic sediments, with Scottish fjords holding a total of 640.7 ± 46 Mt split between 295.6 ± 52 Mt OC and 345.1 ± 39 Mt IC (Smeaton et al., 2017). This value is based upon upscaling the full carbon depth profiles of five Scottish fjords to predict an overall value for the 111 large fjords across Scotland, hence the much larger values relative to the 10 cm surface stock estimates in other studies (Smeaton et al., 2019; 2021a). The sediment of 14 large fjords holds 65% of the total estimate and a medium to very high confidence was placed in 74 of the 111 fjords. Thus, this first order estimate and incorporated uncertainties can be seen as realistic and robust (Smeaton et al., 2017).
Smeaton et al. (2017) conducted the first study to estimate carbon burial for Scottish fjords. The estimated that a total of 31,139 to 40,615 t C yr-1 is being buried in the 111 Scottish fjords annually (Smeaton et al., 2017). A more recent study however has quantified and explored the drivers of OC burial in Scottish fjords using fifteen sediment cores from ten fjords (Smeaton et al., 2021b). Using multiple chronological markers to date the core sediments, the study estimated that, on average, 57.1 ± 10.9 g C m−2 yr−1 accumulates within Scottish fjordic sediments. When combined with the known spatial heterogeneity of sediment type across the fjords (Smeaton et al., 2019; Hunt et al., 2020), it is estimated that Scottish fjords bury 84,000 t of OC annually, which is reported to be equivalent to the whole North Sea sedimentary system (Smeaton et al., 2021b). This is considered a robust estimate and indicates the significant role that fjordic sediments play in long-term blue carbon capture and storage.
An estimation of overall sediment sequestration capacity was attempted within the first national blue carbon audit. The estimate was based upon a review of sediment accumulation rates from northern Europe for OC and data from major deposits to the north-east of the Scottish mainland for biogenic carbonate (Burrows et al., 2014). The study estimated a total sequestration capacity for Scottish sediments of 7.2 Mt OC yr-1 and 0.44 Mt IC yr-1 (Burrows et al., 2014), equating to approximately 28 Mt CO2-eq annually. There is considerable uncertainty in this value due to the paucity of data available to constrain accumulation and burial rate estimates across large areas of the shelf (Smeaton et al., 2021a). Given that sedimentary carbon burial rates vary significantly with sediment type and spatial location, any overall sequestration rate for Scottish coastal and marine sediments should be treated with caution. It is for this reason that an overall sequestration rate has not been calculated, or included as part of this review. It is expected; however, to be considerably lower than most blue carbon habitats, with some parts of the North Sea for instance, experiencing almost no sediment accumulation (Diesing et al., 2021). The best understanding of sedimentary organic carbon burial rates currently exists for Scottish fjords and is well-constrained. Therefore the carbon sequestration rate of 57.1 ± 10.9 g OC m−2 yr−1 is reported within this review as the best available, constrained to Scottish fjords, with the understanding that it is likely to be higher than the average shelf sea burial rate (Smeaton et al., 2021b).
Threats to sedimentary stores
Anthropogenic mobilisation of the sediment, leading to remineralisation of OC (breakdown by microbes converting OC to CO2), could be a significant risk to sedimentary OC stores (Atwood et al., 2020; Legge et al., 2020; Luisetti et al., 2019). Bottom trawling for instance, has been identified as one of the main causes of seabed disturbance within UK waters (Epstein et al., 2022). A study in the Mediterranean assessed the effects of a two-month trawling closure on sedimentary organic matter within a site (Paradis et al., 2021). Cores taken during the trawling season showed ~30% lower OC content, with more labile compounds also being depleted (by 52% to 70%) compared to the sampling conducted on site following the closure (Paradis et al., 2021). Two mechanisms are identified as responsible for removing the OC stock from trawled continental slope sediments. Bottom trawling erodes the organic matter in rich fine-grained sediment, whilst also enhancing the degradation (remineralisation) of more labile compounds (Paradis et al., 2021).
Bottom-trawling activity has been estimated to cause damage equivalent to US$ 0.8 to 6 billion annually for carbon released from UK shelf sea sediments, with one trawl pass estimated to release 0.02 t C ha-1 yr-1 (Luisetti et al., 2019). However, within this particular study, a generic carbon loss rate was applied across the entire of the UK EEZ, which is overly simplistic as sediment type and sedimentary OC content vary significantly here (Smeaton et al., 2021a). A degree of caution is further recommended with the assumption that all disturbed OC is remineralised because it is still not clear what the fate of disturbed carbon is, with partial degradation and subsequent deposition one possibility (Black et al., 2022).
New evidence has emerged regarding the stability of OC within seabed sediments, which augments our understanding of the fate of OC following disturbance. A recent study has highlighted differences in the stability or reactivity of sedimentary OC as a function of location; OC reactivity decreases with distance from the coast (i.e. is more resistant to degradation) (Smeaton and Austin, 2022a). Sedimentary OC on the Scottish continental shelf appears to be relatively stable to degradation (Smeaton and Austin, 2022a), a finding that should be considered within models evaluating the vulnerability of sedimentary carbon to disturbance (Black et al., 2022). That said, there is a high risk of loss of labile OC from sediments found in fjordic and nearshore coastal sediments (Smeaton et al., 2021a; Smeaton and Austin, 2022), which could reduce the potential for these habitats to provide a climate regulation service.
The IC component within seabed sediments is not threatened by disturbance in the same way – calcium carbonate cannot be digested by microbes, and therefore is not degradable via this mechanism (Turrell et al., In Review). However, dislocation of carbonate material due to disturbance is one consequence that could affect in-situ benthos, and therefore biodiversity. Ocean acidification remains an unknown threat that may increase the dissolution of carbonate from the sediments (Doney et al., 2009).
Future research on sediments
Hotspots of OC content have been identified for Scottish sediments within fjords and inshore waters (Smeaton et al., 2021a). These areas may be at greater risk from disturbance and management efforts should perhaps be focused there (Smeaton and Austin, 2022a). A future review could focus on areas where OC hotspots already overlap with fishery management. This could be used to further understand the impacts that restricting trawling has on protecting OC rich sediment stocks and to determine a baseline of these areas. Additionally, the monitoring of vessels under 12 m is needed to compare vessel activity with identified hotspots.
A current evidence gap relates to the lack of reliable burial rate estimates, which would better inform the carbon sequestration capacity of Scottish shelf sea sediments. More data and samples across larger areas of the shelf are required to achieve this. However, priority should perhaps be given to protecting the identified sedimentary hotspots, with a view to understanding their recovery and accretion rates of labile or vulnerable carbon if pressures are removed.
Total sediment depth profiles have only been considered for fjordic sediments, so an evidence gap persists for depth-integrated stocks across coastal and offshore areas in Scotland. Remobilisation of carbon-rich sediments at the seafloor from physical disturbance (e.g. from fishing gear) poses an increased risk to these long-term carbon stores, but this direct disturbance occurs down to specific depths dependent on type of fishing gear and substrate type (Eigaard et al., 2016). Taking into account this longevity and stability, perhaps less focus should be placed on calculating full depth integrated stocks, however analysis of shorter sediment cores that consider the carbon down to the depth associated with fishing activity disturbance warrants further investigation.
Consideration should instead be directed at determining the quality of carbon stored within sediments (Smeaton and Austin, 2022a). Future work is needed to understand how labile the carbon stored within surficial sediments is and the sensitivity of this material to anthropogenic pressures, to better inform management guidance.
Looking across the land-sea interface, management of peatlands may affect how carbon is transported to the sea. A PhD research project ‘'Source to Sea: Soil organic carbon transport from forested environments to coastal sediments' hosted by the University of Stirling is currently assessing the lateral transport of OC from forest soils to sea loch sediments, with potential implications for forestry/catchment management. Perhaps this connectivity approach, known as ‘source-to-sea’, could be applied to a wider range of environments, such as peatlands in the future also. Integrating marine and coastal ecosystems with wider catchment management of was a recommendation for future blue carbon research from the UK’s Climate Change Committee (CCC, 2022). NatureScot are currently supporting a short project to investigate opportunities for more holistic land-sea management in Scotland, which would help to ensure that positive environmental outcomes are maximised across the land-sea interface.
Results and Discussion on sediments
This literature review was compiled with the aim of consolidating currently available information on Scottish blue carbon habitats. Information was presented on the distribution and extent, carbon standing stock, carbon sequestration capacity, threats and blue carbon research needs associated with these marine and coastal habitats. Key findings are presented and discussed in this section, drawing comparisons across habitats and within a broader context.
There are various limitations associated with the data presented in this review. Large uncertainties are mostly associated with limited habitat extent estimates and the lack of Scottish specific carbon rates. Specific evidence gaps are explored in more detail through the individual habitat sections, with a summary presented later in this discussion.
6.1 Wider ecosystem services and functions
Whilst assessing the blue carbon ecosystem service provided by Scottish habitats was the aim of this review, it is important to maintain a holistic approach. Blue carbon is a component of the overall contribution that nature makes to Scotland’s Blue Economy asset base. The relative contribution of blue carbon should be considered in tandem with other ecosystem functions and services. The habitats discussed provide a wealth of other benefits, from coastal defence to supporting biodiversity. Not all of the benefits provided by these habitats can be monetised or quantified as readily as the blue carbon service. A blue carbon focus should not overshadow a habitat’s contribution to other services and instead all aspects should be considered when developing research priorities and policies related to effective decision-making processes and management of Scotland’s coasts and seas. A forthcoming Scottish Blue Carbon Evidence Map aims to consider blue carbon in the broader context of ecosystem functions and services to assess its relative contribution to a broader nature-based solutions approach to managing habitats and species across Scotland.
6.2 Overall habitat summary
Tables 3A (organic) and 3B (inorganic) summarise the carbon storage and sequestration values adopted for this study, derived from the literature. These values are reported with consistent units and applied to known extents of blue carbon habitats in Scottish waters. Brief comments are included to provide more information on the approaches used and any broad limitations. Initial assessments of confidence and data sourced from Scotland are indicated.
A summary of the main pressures associated with the blue carbon habitats has also been compiled, with results presented in Table 4. To further supplement this table, a Feature Activity Sensitivity Tool (FeAST) exists for a range of Scottish features, including several habitats present within this review, and provides a distilled but comprehensive evidence base underpinning the feature’s assessed sensitivity to a range of pressures. Further information on each pressure can also be found within the relevant habitat section of this review.
Table 3A. Summary of the Scottish blue carbon habitat extent and organic carbon contributions. Estimates taken from the literature with sources listed, further information on the methodology, and any caveats are reported in the comments column. UP indicates an unknown parameter.
Habitat | Parameters | Value (± s.d.) | Confidence [a] | Data from Scotland [b] | Source | Comments |
---|---|---|---|---|---|---|
Machair |
Extent (ha) |
11,680 |
High |
Yes |
Burden et al., 2020; Dargie, 2000 |
Total area as measured in 2018, using underlying data from the Sand Dune Vegetation Survey, with individual polygons assigned and interrogated. |
Machair |
OC Density (t C ha-1) |
35.02 |
Medium |
Yes |
Beaumont et al., 2014 |
Carbon from biomass and soil down to 0.15 m, using eight soil samples from six machair sites in the Outer Hebrides. |
Machair |
Total standing stock (t C) |
409,000 |
Medium |
Yes |
Beaumont et al., 2014; Burden et al., 2020 |
Carbon from biomass and soil down to 0.15 m, using eight soil samples from six machair sites in the Outer Hebrides and data from fixed dune grassland. Based on a revised area of 11,680 ha. |
Machair |
Sequestration rate |
34.9 (± 15.7) |
Low |
No |
Beaumont et al., 2014 |
Based on small sample sizes, using the ratio of soil carbon measurements and ecological similarities to sand dune grasslands instead. |
Machair |
Sequestration capacity |
4,076 |
Low |
No |
This review |
– |
Sand dunes |
Extent (ha) |
15,022 |
Low |
Yes |
HabMoS database; Dargie, 2000; Angus, 2011
|
Data taken from Sand Dune Vegetation Survey and reclassified / standardised to Annex I habitat. (Includes H2110, H2120, H2130, H2140, H2150, H2170, H2190) |
Sand dunes |
OC Density (t C ha-1) |
7.17 – 63.7 (soils)
5.12 - 5.91 (above ground vegetation)
10.05 - 16.4 (below ground roots) |
Low |
No |
Beaumont et al., 2014 |
Range estimates for soils (0.15 m depth) in mobile dunes (7.17), fixed dunes (33.29) and dune slacks (63.7). Range estimates for above ground vegetation in fixed dunes (5.12), dune slacks (5.43) and mobile dunes (5.91). Range estimates for below ground root stock in mobile dunes (10.05), fixed dunes (10.74) and dune slacks (16.4) |
Sand dunes |
Total standing stock (t C) |
752,954
504,895 (soil) 78,581 (above ground vegetation) 169,478 (below ground roots) |
Low |
No |
Beaumont et al., 2014 |
From biomass above ground, below ground in roots and in soil down to 0.15 m taken from 11 dune systems around England and Wales. |
Sand dunes |
Sequestration rate |
60 |
Medium |
No |
Jones et al., 2008 |
Utilising the proportion of dry dune vegetation (90%) to dune slack (10%), a rate was calculated based on samples from Newborough Warren, North Wales, UK. |
Sand dunes |
Sequestration capacity |
9,013 |
Low |
No |
This review |
Taking the proportional sequestration rate and the areal extent of habitat gives estimated annual sequestration capacity. |
Saltmarsh |
Extent (ha) |
5,840 |
Medium |
Yes |
Haynes, 2016 |
Refers to saltmarsh vegetation mapped between 2010 and 2012. |
Saltmarsh |
OC Density (t C ha-1) |
63.1 (± 15.6) |
High |
Yes |
Smeaton et al., 2022 |
Average carbon stock estimated for the standardised top 10 cm of Scottish saltmarsh soils by Smeaton et al., (2022). OC density increases with depth due to soil compaction. |
Saltmarsh |
Total standing stock (t C) |
1,149,281 +/- 250,031
368,000 (± 91,000) (top 10 cm of soil) |
High |
Yes |
Miller et al., 2023
Smeaton et al., 2022
|
Carbon stock estimated for combined components of Scotland's 240 mapped saltmarshes, including above and below ground biomass and full depth of saltmarsh soil units (fibrous peat, humified peat and transitional). Carbon stock in standardised 0.1 m depth of soil for Scotland's saltmarsh. |
Saltmarsh |
Sequestration rate |
87.45 |
High |
Yes |
Miller et al., 2023 |
Average value for Scottish saltmarshes estimated from mud-rich and sand-rich saltmarsh sites and accounting for the upper and lower marsh zones using multiple chronological markers. |
Saltmarsh |
Sequestration capacity |
4,385 (± 481) |
Medium |
Yes |
Miller et al., 2023 |
Annual OC accumulation estimated for Scottish saltmarshes by applying mean rates from the upper and lower marsh zones from three representative saltmarsh sites. 85% of the accumulated OC is from terrestrial sources. |
Seagrass beds |
Extent (ha) |
1,504 |
Medium |
Yes |
This review |
Extracted data from GeMS and combined with survey estimates of Udale Bay and Nigg Bay (Marine Scotland, 2020). |
Seagrass beds |
OC Density (t C ha-1) |
110 |
High |
Yes |
Potouroglou et al., 2021 |
Geological OC (top 0.5 m) from seven Scottish estuaries, extrapolated to 1 m. Large sample size and stratified cores improve confidence. |
Seagrass beds |
Total standing stock (t C) |
165,440 |
Medium |
Yes |
This review |
Geological stock. |
Seagrass beds |
Sequestration rate |
67.91 |
Medium |
No |
Lima, 2020 |
Based on six intertidal seagrass meadows within the Solent, southern England. |
Seagrass beds |
Sequestration capacity |
1,021 |
Medium |
No |
This review |
– |
Kelp beds |
Extent (ha) |
374,700 |
Medium |
Yes |
Burrows et al., 2018 |
Derived from a statistical model fitted to MNCR data collected in Scotland (1980-2005), representing areas where Laminaria biomass density is likely >2 kg m-2. Equates to a biomass estimate of 15.54 Mt. |
Kelp beds |
OC Density (t C ha-1) |
~2 – 9.76 |
Medium |
Yes |
King et al., 2020; Pessarrodona et al., 2018 |
Range based on studies from Scottish sites, based on L. hyperborea and L. digitata. All studies discussed collected data from kelp beds at relatively shallow depths (<7m). This likely overestimates carbon when applying these values across all sites |
Kelp beds |
Total standing stock (t C) |
~600,000 |
Low |
Yes |
This review |
Based upon biomass estimate for L. hyperborea and underlying assumptions whereby dry weight is 15% of fresh weight, with a 30% carbon content of dry matter. |
Kelp beds |
Production rate |
378 |
Medium |
Yes |
Smale et al., 2020 |
No local sequestration occurs, so production rate is given. An average from Scottish studies. |
Kelp beds |
Production capacity |
1,416,000 |
Low |
Yes |
This review |
Extent * Production rate give estimated production capacity. |
Sediment |
Extent (ha) |
47,666,600 |
– |
Yes |
Smeaton et al., 2021a |
Represents Scottish sector of the UK EEZ (476,666 km2). |
Sediment |
Area-specific stock (t C ha-1) |
7.5 (7.8 – 15.03) |
Medium |
Yes |
Smeaton et al., 2021a |
Average OC density for Scottish seabed calculated simply using total OC stock and total extent (for 0.1 m depth). Range of mean OC densities for the different seabed regions also presented to highlight spatial variation dependent on proximity to shore (slope and deep sea (9.59), continental shelf (7.8), coastal and inshore (10.6), fjords (15.03)). |
Sediment |
Total stock (t C) |
2,630.3 x 106
356.5 (± 72.2) x 106 OC; (± 156.3) x 106 IC |
Medium |
Yes |
Smeaton et al., 2021a |
An estimate of standing stock of OC and IC (top 0.1 m) within Scottish adjacent waters using a spatial modelling approach. |
Sediment |
Sequestration rate |
57.1 ± 10.9 |
Medium |
Yes |
Smeaton et al., 2021b |
This value is based on OC burial rates within Scottish fjords. There are very limited data for C sequestration rates for the wider shelf seas; however rates are likely to be higher within fjords relative to the shelf. |
Sediment |
Sequestration capacity |
UP |
- |
- |
- |
No estimate provided due to a lack of data to constrain burial rates across large areas of the shelf. Sequestration rates vary considerably spatially and with sediment type. |
[a] Indicates the level of confidence in estimates.
[b] Indicates whether the underlying data are from Scottish sites. Extent data are inherently based on information for Scottish sites.
Table 3B. Summary of the Scottish calcifying aggregation (supporting blue carbon habitat) extents and inorganic carbon contributions. Estimates taken from the literature with sources listed, further information on the methodology, and any caveats are reported in the comments column. UP indicates an unknown parameter.
Habitat | Parameters | Value (± s.d.) | Confidence [a] | Data from Scotland [b] | Source | Comments |
---|---|---|---|---|---|---|
Maerl beds |
Extent (ha) |
3143 |
Medium |
Yes |
This review |
Extracted data from GeMS. |
Maerl beds |
IC Density (t IC ha-1) |
104 |
Low |
Yes |
Burrows et al., 2014 |
IC density standardised to 0.1 m bed thickness. Average bed thickness for maerl is 0.6 m. |
Maerl beds |
Total standing stock (t IC) |
1,960,000 |
Low |
Yes |
This review |
Total stock estimated for extent of maerls beds and assuming an average bed depth of 0.6 m. |
Maerl beds |
IC production rate |
57.4 |
Low |
No |
Bosence & Wilson, 2003; Martin, et al., 2007; Savini, et al., 2012 |
Mean IC rate for Lithothamnion glaciale and Phymatolithon calcareum from European studies. |
Maerl beds |
IC production capacity |
541 |
Low |
No |
This review |
Based upon applying a blanket 30% live maerl status, with this average calculated from 103 records in Marine Recorder. |
Cold-water coral reefs (Lophelia pertusa) |
Extent (ha) |
684 |
Low |
Yes |
Burrows et al., 2014 |
Includes Darwin Mounds and Mingulay Reef Complex, as they are the only well-defined Scottish coral reefs. Value is likely an underestimate. |
Cold-water coral reefs (Lophelia pertusa) |
IC Density (t IC ha-1) |
37.44 |
Low |
Yes |
Burrows et al., 2014 |
IC density based on estimate of 312 kg m-3 and standardised to 0.1 m reef thickness. Average reef thickness in Shetland is 0.2 m. |
Cold-water coral reefs (Lophelia pertusa) |
Total standing stock (t IC) |
125,500 |
Low |
Yes |
Burrows et al., 2014 |
Assumes that coral accounts for 33% of the reef mound volume. |
Cold-water coral reefs (Lophelia pertusa) |
IC production rate |
35 |
Low |
Yes |
Burrows et al., 2014; Douarin et al., 2014 |
IC sequestration rate for Mingulay based on simplifying assumptions, likely lower for the Darwin Mounds as it is a less productive area. |
Cold-water coral reefs (Lophelia pertusa) |
IC production capacity |
239.4 |
Low |
Yes |
This review |
– |
Serpulid aggregations (Serpula vermicularis) |
Extent (ha) |
12.9 |
Low |
Yes |
Dodd et al., 2009; Moore et al., 2009 |
Total Scottish known extent estimated from reef area and coverage, does not include reported declines. |
Serpulid aggregations (Serpula vermicularis) |
IC Density (t IC ha-1) |
11.5 |
Low |
Yes |
Burrows et al., 2014 |
Based on an estimated density of 28,644 g CaCO3 m-2, and standardised to a 0.1 m tube height. Tube clusters vary in height between 0.3 m and 0.6 m in Loch Teacuis and Loch Creran respectively. |
Serpulid aggregations (Serpula vermicularis) |
Total standing stock (t IC) |
~1000 |
Low |
Yes |
Burrows et al., 2014 |
Standing stock varies from 859 – 1247 t IC depending on the reef height assumed for Loch Creran, |
Serpulid aggregations (Serpula vermicularis) |
IC production rate |
420 |
Low |
Yes |
Burrows et al., 2014 |
Based on estimates from Loch Creran and assuming a similar reef morphology to Galeolaria hystrix in New Zealand. Refers to carbon production and likely an overestimate of sequestration. |
Serpulid aggregations (Serpula vermicularis) |
IC production capacity |
54.2 |
Low |
Yes |
This review |
– |
Flame shell (Limaria hians) |
Extent (ha) |
2,269 |
Medium |
Yes |
Burrows et al., 2014; Moore et al., 2013, 2018, 2020; Porter et al., 2020 |
Includes extent of known Scottish beds and predictive modelling, with the values representing >90% to >50% cover, and some representing >10% cover. |
Flame shell (Limaria hians) |
IC Density (t IC ha-1) |
0.3 |
Medium |
Yes |
Porter et al., 2020 |
Estimated from Orkney flame shell beds for a nest thickness of 0.21 m. The reported value had been standardised to 0.1 m depth. |
Flame shell (Limaria hians) |
Total standing stock (t C) |
1,740 t IC 6,223 t OC
|
Medium |
Yes |
Burrows et al., 2014; Porter et al., 2020 |
Uses a shell carbon composition of 0.913 g per individual; 0.72 g OC and 0.197 g IC (Porter et al., 2020) and extents of beds at surveyed sites. |
Flame shell (Limaria hians) |
IC production rate |
UP |
– |
– |
– |
– |
Flame shell (Limaria hians) |
IC production capacity |
UP |
– |
– |
– |
– |
Horse mussel beds (Modiolus modiolus) |
Extent (ha) |
4,238 |
Low |
Yes |
Hirst et al., 2012 |
Includes extent of modelled beds in Orkney and the bed off Noss Head, Caithness. |
Horse mussel beds (Modiolus modiolus) |
IC Density (t IC ha-1) |
5.33 |
Low |
Yes |
Porter et al., 2020; Collins, 1986; |
Samples from Cava Island and the Firth of Lorn for IC. A density of 40 t IC ha-1 was estimated for an average bed thickness of 0.75 m (Porter et al., 2020). Reported value has been standardised to 0.1 m thickness. Assumed a uniform density and a limited number of sites were assessed. |
Horse mussel beds (Modiolus modiolus) |
Total standing stock (t C) |
169,520 |
Low |
Yes |
This review |
Value for the assumed full depth (0.75 m) of Scottish extent – likely to be an underestimate due to lack of modelling >50 m depth. |
Horse mussel beds (Modiolus modiolus) |
IC production rate |
40 |
Low |
Yes |
Collins, 1986 |
From the Firth of Lorn. Caution in applying this value across Scotland as other sites have not been sampled. |
Horse mussel beds (Modiolus modiolus) |
IC production capacity |
1,695 |
Low |
Yes |
This review |
– |
Blue mussel beds (Mytilus edulis) |
Extent (ha) |
>400 |
Low |
Yes |
SNH, 2018c |
Extent mapping for this habitat in Scotland is lacking. Largest known bed is the Firth of Tay at >400 ha. |
Blue mussel beds (Mytilus edulis) |
IC Density (t IC ha-1) |
UP |
– |
– |
– |
– |
Blue mussel beds (Mytilus edulis) |
Total standing stock (t C) |
UP |
– |
– |
– |
– |
Blue mussel beds (Mytilus edulis) |
IC production rate |
62.9 |
Medium |
No |
Jansen & van den Bogaart, 2020 |
Values depended on the method used to calculate overall sequestration (how respiration and / or calcification were incorporated) and whether the mussels were sheltered or exposed. |
Blue mussel beds (Mytilus edulis) |
IC production capacity |
UP |
– |
– |
– |
– |
Native oyster (Ostrea edulis) |
Extent (ha) |
UP |
– |
Yes |
– |
– |
Native oyster (Ostrea edulis) |
IC Density (t IC ha-1) |
UP |
– |
– |
– |
– |
Native oyster (Ostrea edulis) |
Total standing stock (t C) |
UP |
– |
– |
– |
– |
Native oyster (Ostrea edulis) |
IC production rate |
UP |
– |
– |
– |
– |
Native oyster (Ostrea edulis) |
IC production capacity |
UP |
– |
– |
– |
– |
Brittlestar beds (Ophiothrix fragilis) |
Extent (ha) |
UP |
– |
Yes |
– |
– |
Brittlestar beds (Ophiothrix fragilis) |
IC Density (t IC ha-1) |
0.79 |
Low |
Yes |
Porter et al., 2020 |
Value is for IC only, with samples taken from a bed north of Cava Island. Low confidence in these values due to a small sample size (n=5) although this is a similar value to that reported in the Dover Strait, 0.66 t IC ha-1 |
Brittlestar beds (Ophiothrix fragilis) |
Total standing stock (t C) |
UP |
– |
– |
– |
– |
Brittlestar beds (Ophiothrix fragilis) |
IC production rate |
81.8 |
Low |
No |
Migne et al., 1998 |
IC sequestration rate calculated for the Dover Strait O. fragilis bed. |
Brittlestar beds (Ophiothrix fragilis) |
IC production capacity |
UP |
– |
– |
– |
– |
Bryozoan thickets (Flustra foliacea) |
Extent (ha) |
UP |
– |
Yes |
– |
– |
Bryozoan thickets (Flustra foliacea) |
IC Density (t IC ha-1) |
0.3 |
Low |
Yes |
Porter et al., 2020 |
Value is for IC only (total carbon density estimated to be 5.03 t C ha-1); Samples taken from F. foliacea colonies in Orkney, does not include other Bryozoa species. Unknown sample size. |
Bryozoan thickets (Flustra foliacea) |
Total standing stock (t C) |
UP |
– |
– |
– |
– |
Bryozoan thickets (Flustra foliacea) |
IC production rate |
UP |
– |
– |
– |
– |
Bryozoan thickets (Flustra foliacea) |
IC production capacity |
UP |
– |
– |
– |
– |
[a] Indicates the level of confidence in estimates.
[b] Indicates whether the underlying data are from Scottish sites. Extent data are inherently based on information for Scottish sites.
Table 4. Summary of the potential pressures associated with each blue carbon habitat, relevant to Scotland. Further sensitivity assessments for the marine habitat PMFs can be found within the Feature Activity Sensitivity Tool (FeAST).
Habitat | Pressure | Source |
---|---|---|
Machair |
Grassland improvement; grazing; increased storm frequency/severity; sea level rise |
Angus et al., 2011; Angus and Hansom, 2004; Angus and Rennie, 2014 |
Sand dunes |
Coastal erosion; changing rainfall patterns; grazing; sea level rise |
Beaumont et al., 2014; Burden et al., 2020 |
Saltmarsh |
Grazing; nutrient enrichment; pollution |
Burrows et al., 2014; Morris and Jensen, 1998 |
Seagrass beds |
Changes in water flow; increased sea temperature; increased storm frequency/severity; INNS; nutrient enrichment; physical disturbance; sea level rise; smothering |
d’Avack et al., 2014; Grech et al., 2011; Marbà and Duarte, 2009; Orth et al., 2006; Preen and Marsh, 1995; Yan et al., 2019 |
Kelp beds |
Harvesting potential; increased sea temperature; increased storm frequency/severity |
Burrows et al., 2014; Fernández, 2011; Pessarrodona et al., 2018; Smale et al., 2013 |
Maerl beds |
Changes in water flow; increased benthic temperature; INNS; ocean acidification; organic enrichment/pollution; physical disturbance; sedimentation |
Burrows et al., 2014; Hall-Spencer et al., 2006; Hall-Spencer and Moore, 2000a; Kamenos et al., 2013; Martin and Hall-Spencer, 2017; Simon-Nutbrown et al., 2020 |
Cold-water coral reefs (Lophelia pertusa) |
Increased turbidity; ocean acidification; physical disturbance; suspended solids |
Büscher et al., 2017; Hall-Spencer and Stehfest, 2009; Hourigan, 2009; NatureScot, 2019 |
Serpulid aggregations (Serpula vermicularis) |
Changes in water flow; increased storm frequency/severity; INNS; ocean acidification; physical disturbance |
Burrows et al., 2014; Smith et al., 2013; SNH, 2018b |
Flame shell |
INNS; ocean acidification; physical disturbance |
Burrows et al., 2014; Hall-Spencer and Moore, 2000b |
Horse mussel beds (Modiolus modiolus) |
Increased sea temperature; INNS; ocean acidification; physical disturbance |
Fitzer et al., 2015; Gormley et al., 2013; Hutchison et al. 2016 |
Blue mussel beds (Mytilus edulis) |
Increased sea temperature; INNS; ocean acidification; physical disturbance; sea level rise |
Fitzer et al., 2015; Mainwaring et al., 2014; SNH, 2018c; Burrows et al., 2017; Baden et al., 2020 |
Native oyster (Ostrea edulis) |
Changes in water flow; illegal harvesting; increased sea temperature; increased storm frequency/severity; INNS; ocean acidification; organic enrichment; physical disturbance; pollution |
Lancaster et al., 2014; Lemasson et al., 2017; Mazik et al., 2015; SNH, 2018d |
Brittlestar beds (Ophiothrix fragilis) |
Increased sedimentation; ocean acidification; physical disturbance |
Aronson, 1989; De-Bastos and Hill, 2016; Dupont et al., 2008 |
Bryozoan thickets (Flustra foliacea) |
Ocean acidification; physical disturbance; increased sedimentation |
Jennings and Kaiser, 1998; Smith, 2014; Woods et al., 2012 |
Sediment |
Ocean acidification; physical disturbance |
Doney et al., 2009; Luisetti et al., 2019; Epstein et al., 2022 |
6.3 Carbon storage
Surficial sediments hold an estimated 357 (± 722) Mt OC and 2,265 (± 156) Mt IC (Table 3A), comprising the vast majority of blue carbon stored within the Scottish environment (Figure 2). This is considered a robust estimate, with high confidence in the values.
The cumulative blue carbon stored within Scottish coastal vegetated and nearshore habitats is modest compared to the sedimentary store, estimated to be ~3.1 Mt OC, however these habitats are essential for safeguarding biodiversity and provide multiple stacked benefits (Figure 2). Saltmarsh makes up 50% of the coastal habitats’ standing stock and kelp beds comprise over 75% of the carbon standing stocks in nearshore habitats. This is due to the high productivity of kelp relative to seagrass, which is held within living biomass, however unlike seagrass, kelp cannot transfer OC below-ground because of a lack of a rook system.
The calcifying aggregations, defined within this review as supporting blue carbon habitats, are estimated to store approximately 2.3 Mt IC (Figure 2); although CO2 is released during calcification, the carbonate skeletons of many of the longer-lived habitats provide a long-term (> 100 years) store of the remaining carbon. They are also essential components of a healthy and fully functioning marine environment. Standardised to a 0.1 m depth, maerl appears to make the highest contributions to IC for the calcifying aggregations, estimated to have an IC density of 104 t C ha-1 (Table 2B) and a standing stock of ~1.96 Mt IC, forming almost 90% of the total IC standing stock. Whilst there are uncertainties arising from limited observations of maerl bed thickness used in IC calculations and the potential facilitation service of OC burial in underlying sediments, these estimates highlight the importance of Scottish maerl beds in particular to blue carbon storage. This review has attempted to standardise the IC density for calcifying aggregations to 0.1 m depth to allow a crude comparison between different habitats. It is acknowledged that these values have high uncertainty not least because of the complex 3D structures of many of these habitats that are not uniform in space.
The amount of carbon reported within Scottish sediments is an underestimate, as only the top 0.1 m is considered. However, calculating robust full depth integrated stock estimates is likely not a priority as the carbon in deep sediments is sequestered over the long-term and considered relatively stable (Smeaton and Austin, 2022). Remobilisation of organic rich sediments at the seafloor from physical disturbance poses an increased risk to these long-term carbon stores, but this direct disturbance likely only occurs down to a specific depth (Eigaard et al., 2016; Black et al., 2022). Further consideration should therefore be targeted at quantifying the carbon associated with these depths, using multicores for sampling instead of surface grabs for instance, to better understand the impacts of pressures on carbon stores below the surface, and therefore the management implications for such activities.
Large uncertainties also persist with the standing stock estimates of the biological blue carbon habitats (with the exception of saltmarsh) due to a lack of national-scale, contemporary mapping data, as well as spatially limited carbon content and sequestration data. Recent research on Scottish saltmarshes has helped to understand variability in carbon storage and accumulation based on saltmarsh type and zone (Miller et al., 2023). This information allows more nuanced estimates of carbon stocks and defined hotspots, and highlights similar spatial knowledge gaps that exist for other habitats. Gaps in our understanding of methane emissions from saltmarsh persist however.
6.4 Carbon sequestration
Whilst it is expected that the total sequestration capacity for Scottish sediments would be considerably larger than all blue carbon habitats combined (Burrows et al., 2014), the sequestration rate is likely modest in comparison (Figure 3). The sequestration rate of 57.1 ± 10.9 g C m-2 yr-1 reported (Table 3A) has been measured within Scottish fjords, which are hotspots for carbon burial due to their proximity to land and glacially over-deepened basins (Smeaton et al., 2021b). It is not likely to be representative of the wider shelf and as such, would have large associated uncertainties if used beyond the inshore environment. An overall sequestration capacity has thus not been provided as this varies considerably with distance from land and sediment type and there is a significant lack of data across large areas of the Scottish shelf to constrain any estimates.
For the vegetated blue carbon habitats, excluding kelp, this review estimates that they sequester a combined 18,500 t C yr-1 (~0.02 Mt C yr-1) (Figure 3). This value holds large uncertainties arising from the low confidence in extent estimates and lack of robust Scottish-specific sequestration rates for many of the habitats. Kelp are considered an important carbon donor to neighbouring habitats (Krause-Jensen and Duarte, 2016), but since they grow on rocky substrates, they are not able to sequester carbon locally. In Table 3A, this is acknowledged by providing the production rate and production capacity for kelp instead. With a significant production rate, kelp have a production capacity 70 times higher than the combined sequestration capacity of saltmarsh, sand dunes, seagrass and machair. This highlights the importance of this habitat in its role as a carbon donor and as a potential pathway for atmospheric carbon drawdown.
The calcifying aggregations, where data are available, are estimated to produce ~ 2,500 t IC annually via carbonate production (Figure 4). This is a likely underestimate due to a lack of rate data for many of the habitats within this review.
This review has identified the most productive blue carbon habitats as being, kelp (378 g C m-2 yr-1), saltmarsh (87.45 g C m-2 yr-1), and seagrass (67.91 g C m-2 yr-1) (Figure 3). Kelp do not sequester this carbon locally with the proportion sequestered elsewhere (i.e., in adjacent sediments) largely unknown. The rate for saltmarsh is based on a recent spatially representative dataset for Scottish saltmarsh systems and is considered robust, however, the rate for seagrass is based on studies outside of Scotland and may not be representative of Scottish systems. Serpulid aggregations are estimated to produce 420 g IC m-2 yr-1, not accounting for any CO2 emissions. Although this is a relatively high IC production rate, due to limited extent in Scottish waters their contribution to overall IC production capacity is relatively small (Figure 4).
In terms of total carbon sequestration capacity, Scottish sand dunes have the highest estimate of ~9,000 t C yr-1. However, this value is based on an extent estimate from the Scottish Sand Dune Vegetation Survey conducted over 20 years’ ago (Dargie, 2000) and as dune systems are highly dynamic, this extent has likely changed. Additionally, the sequestration rate used in this calculation was based on samples from one site in Wales (Jones et al., 2008), which may not be representative of Scottish dune systems as a variety of factors, such as vegetation type and hydrology, determine their characteristics. It is not recommended that sand dunes are managed for their blue carbon service specifically because dune systems require dynamic condition to remain functional (Van der Biest et al., 2017). While over-stabilisation of dune systems may increase the carbon sequestration and storage potential, however it also affects their natural function, reducing their ability to adapt to changing climatic conditions and thus decreasing their resilience (Pye, Blott & Howe, 2014).
6.5 Evidence gaps
Numerous evidence gaps remain for Scotland’s blue carbon habitats. Further research is needed to improve understanding of how carbon sequestration in saltmarshes varies, for instance according to season, condition, and grazing management, as well as the net sequestration status accounting for methane emissions. Additionally, the blue carbon service provided by mudflats should be investigated because these two habitats are naturally interchangeable at the boundaries (Ladd, 2021). The perturbation of existing saltmarsh carbon stores and how they may be impacted by increasing pressures warrants further research. Given the occurrence of overgrazed saltmarsh on the west coast (Site Condition Monitoring Assessments carried out by NatureScot) and lack of Scottish specific research, assessments into the impacts of different grazing regimes on the Scottish saltmarsh blue carbon store need to be undertaken. This habitat requires further focus, from a blue carbon and broader perspective, to help improve knowledge and better inform management decisions relating to climate adaptation and restoration.
Given Scottish sand dunes appear to have the highest potential sequestration capacity, focus should be on determining the extent and direction of change since the last national survey and ascertaining estimates for Scottish specific sequestration rates within each zone. This research need could partially be addressed through utilising data from the ongoing Dynamic Coast project to improve upon the Scottish estimate of extent; however, loss of extent is a natural phenomenon that should be considered in extent accounting. This potential overlap will be explored further before prioritising recommendations for a sand dune survey, which would be costly and only provide a snapshot of this dynamic system. Further work is needed to understand the pH and mineralogy of Scottish sites, and how this influences the blue carbon service. Researching how different grazing regimes impact Scottish dune systems, their condition, and their blue carbon service is another recommendation. With restoration of sand dunes a possibility, further work should also assess the full range of ecosystem service potential of restoring sand dunes from conifer plantations, including from a coastal adaptation perspective. However as noted previously, management of sand dunes for their carbon service alone, which may favour over stabilisation, is not a recommended action.
As seagrasses have a relatively high sequestration rate and there is a growing interest in restoration projects within Scotland, current evidence gaps should be considered. Global carbon sequestration rates are dominated by calculations based on two main species – P. oceanica and C. nodosa – with a regional bias towards Mediterranean and Australian sites (Fourqurean et al., 2012). Further research on Zostera spp. within Scottish meadows is required, to provide a more reliable estimate for the carbon sequestration rate. Further research is needed to determine how different types of Scottish seagrass beds – dense Z. marina beds compared to patchy Z. noltii beds; intertidal versus subtidal – influence the blue carbon service. Seasonal fluctuations also need to be investigated, with suggestions for sampling to be conducted during multiple seasons. As restoration work progresses, research should also be targeted at comparing the carbon and biodiversity services provided by natural and restored seagrass beds with ongoing monitoring from a baseline state.
Kelp are highly productive habitats and important blue carbon donors. Research to understand the long-term contributions that these habitats make to blue carbon stores is essential to understand their actual mitigation potential. Tracing experiments using eDNA or stable isotope techniques can help to address this gap in knowledge (Queirós et al., 2019). Interest in the potential of macroalgal aquaculture is increasing as a viable means of reducing atmospheric CO2 and reduction of emissions from industries such as agriculture that rely heavily on fossil fuels as energy sources (Duarte et al., 2021).
It is clear that the supporting blue carbon habitats, calcifying aggregations, are understood the least. Whilst other habitats may have low-medium confidence placed in some of their parameters, half of the calcifying habitats have at least one unknown variable. In particular, blue mussel beds, native oysters and bryozoan communities have limited known parameters from the literature. Current research is targeting this lack of understanding for blue mussel and native oyster beds with a recent PhD project ‘The Blue Carbon of Shellfish Beds: Understanding the vaults of biogenic reefs (O. edulis, M. modiolus and M. edulis)’ hosted by Heriot Watt aiming to improve mapping of Scottish beds and quantify their contribution to the blue carbon service. This has the potential to provide baseline estimates for some of the unknown parameters for these habitats. The aim of this review is to present an initial sift of the literature, reporting on information currently published. Any key emerging findings from ongoing research will be explored further in a subsequent report. In the case of Bryozoa and brittlestar beds, the limited research could be related to the lack of conservation designation status. Given the relative pressures on other protected habitats and Priority Marine Features (PMFs), these habitats have limited drivers for prioritisation.
A large omission within this review is an estimate of sequestration rate and annual capacity for shelf sediments. This is due to extremely poor spatial data for sediment and OC burial rates across much of the Scottish shelf. This is a difficult evidence gap to fill due to high costs of sampling and dating analysis given the large extent to sample. However, it is an important evident gap to fill to understand the role of shelf sediments in climate change mitigation.
6.6 Recurring pressures
Climate-driven pressures were a key recurring threat across all habitats (Table 4). Ocean acidification was particularly prevalent, with this predicted to impact all habitats considered under calcifying aggregations. Over longer timescales, ocean acidification may lead to the chemical and physical breakdown of these deposits, with potential loss of habitat and ecosystem function (Burrows et al., 2014; Macreadie et al., 2017; Hennige et al., 2020). Sedimentary stores may also be impacted by ocean acidification, leading to the dissolution of carbonate from the sediments (Doney et al., 2009). The remaining blue carbon habitats – coastal, seagrass and kelp – face climatic pressures including sea level rise, increased sea temperature, increased storm frequency/severity and changing rainfall patterns.
The pressures linked with the changing climate are unmanageable on local scales. In the case of sea level, even if global net zero CO2 emissions are achieved by 2100, RSLR is predicted to continue for centuries (Wong et al., 2014). Focus should instead be targeted towards how climate-driven factors work in tandem with other anthropogenic pressures, with the aim of reducing these potentially manageable pressures.
In regards to directly manageable pressures, physical disturbance was prevalent across the majority of habitats considered (Table 4). Bottom-contact fishing activity is considered within this pressure definition. The PMFs included in this review that have been identified as most vulnerable to bottom-contact fishing are: blue mussel beds, native oyster, cold-water coral reefs; flame shell beds; horse mussel beds; serpulid aggregations; maerl beds and seagrass beds (Marine Scotland, 2019; FeAST). This pressure is also relevant and potentially significant when considering brittlestar beds and sediment stores.
Bottom trawling has been identified as one of the main causes of seabed disturbance within UK waters, with one trawl pass estimated to release 0.02 t C ha-1 yr-1 (Luisetti et al., 2019). This average is over-simplistic, as it does not take into account how OC content varies with sediment type and the quality of the organic matter (Smeaton and Austin, 2022). As muddy sediments are generally higher in OC content (Diesing et al., 2017; Hunt et al., 2020), these areas may be at greater risk from disturbance and management efforts should be focused there (Smeaton et al., 2021a). Methods to improve spatial understanding of the distribution of sedimentary OC at regional scales (Hunt et al., 2021; Pace et al., 2021) and the vulnerability of OC (Black et al., 2022) can help to prioritise areas for seabed management.
Overgrazing on saltmarsh is a known pressure, particularly on the west coast of Scotland. It is unknown how this affects carbon stocks and sequestration however, there are likely to be complex feedback mechanisms. Methane emissions could also be affected by grazing levels that over-compact the soil (Gregg et al., 2021). Other pressures exist, as reported by the previous habitat sections and outlined in Table 4.
6.7 Wider context
Although the total carbon stored by coastal and marine sediments represents a larger contribution than that stored by Scottish forests or peatlands, the majority (86%) of this is inorganic (Figure 2). The organic proportion of the total stock can be compared directly to the carbon biomass and soil stocks of terrestrial forests and peatlands, which are also organic. Forests in Scotland are estimated to store 560 Mt of carbon (Forestry Statistics, 2021) taking into account tree biomass, litter, deadwood and soil carbon (1 m depth), with an estimated stock of 2,000 Mt OC in Scottish peatlands (Aitkenhead & Coull, 2019). While there is a greater total carbon stock held within blue carbon habitats, the lower proportion of organic material highlights a reduced role in climate change mitigation potential compared to terrestrial habitats, a result of a relatively lower spatial extent of vegetated blue carbon habitats.
Whilst Scottish peatlands have a significantly larger organic stock compared to the combined blue carbon habitats, the average sequestration rate of peatlands is considerably lower (Figure 2). Yu et al. (2009) calculated a mean rate of carbon sequestration of 18 g C m-2 yr-1 for northern peatlands and a study by Ratcliffe et al. (2018) extrapolated an average value of 16.3 g C m-2 yr-1 from cores sampled from peatlands in Flow Country, Scotland. The condition of the peatland influences the rate of sequestration with a healthy peatland estimated to sequester up to 27 g C m-2 yr-1 (Evans et al., 2017; Aitkenhead and Coull, 2019). These values are lower than the sequestration rates reported in Figure 3 for Scottish blue carbon habitats. Additionally, degraded peatland can also act as a source of greenhouse gas emissions, with climate change further exacerbating potential losses of carbon from peatland (Ferretto et al., 2019).
In the wider UK context, Scottish blue carbon stores are greater than that those in the Welsh marine environment. A recent assessment by NRW found 113 Mt of carbon stored within Welsh surficial sediments (Armstrong et al., 2020), while another study estimated a more modest total of carbon stock of ~47 Mt C comprising 21 Mt OC and 26 Mt IC over 32,850 km2 of seabed (Smeaton et al., 2021a). This is compared to the value of ~2,600 Mt C reported for Scottish sediments (Smeaton et al., 2021a). For Welsh habitats, a combined standing stock of 0.4 Mt C was reported (Armstrong et al., 2020) compared to the ~2.3 Mt OC estimated for vegetated Scottish habitats in this review. The total sequestration capacity, ~0.03 Mt C yr-1 is estimated for Welsh marine habitats including sediments (Armstrong et al., 2020) compared to a rate of ~0.02 Mt C yr-1 found for Scottish blue carbon habitats, excluding sediments for which the sequestration rate data are too limited to constrain estimates. However, the total Scottish sequestration capacity would be expected to be significantly higher with the inclusion of sediments given the high sequestration rates for fjords, and the much larger area of the Scottish EEZ.
Natural England has recently carried out a review of the carbon storage and sequestration of blue carbon habitats finding similar data limitations in terms of habitat extents, geographical spread of data, and the English-specific habitat data to estimate stocks and sequestration rates (Gregg et al., 2021). Smeaton et al. (2021a) estimate a total surface sediment stock of ~420 Mt C, comprising 142 Mt OC and 277 Mt IC within England’s Secretary of State waters, which cover an area of 227,580 km2, approximately half the size of Scotland’s seabed area. The areal extent of England’s saltmarshes is estimated to be 336 km2 and holds 1.6 Mt OC in the surficial soils; this is compared to Scotland’s 0.37 Mt OC over 58 km2 and Wales’s 0.35 Mt OC over 58 km2 (Smeaton et al., 2022b). Values for other blue carbon habitat stocks and sequestration rates for England are provided within Gregg et al.’s report (2022). An assessment of the blue carbon resources within Jersey’s territorial seas has also been recently completed which estimates that Jersey’s seas have approximately 100,000 t OC stored within living plants and animals and a further 1,200 t C yr-1 are sequestered annually (Chambers et al., 2022a). Approximately 12 Mt IC exist within calcifying aggregations and seabed sediment as shell (Chambers et al., 2022a). National audits for blue carbon can inform regional spatial planning for improved coastal and marine management and national carbon accounting. The UK Blue Carbon Forum aims to address the role of blue carbon in mitigating the current ecological and climate emergency. It will aid collaboration between the UK nations to ensure standardised approaches to blue carbon science are adopted, data are shared in a meaningful way, and will support the incorporation of blue carbon into the wider UK’s climate mitigation strategy.
With respect to previous national assessments, this review has updated values compared to the prior assessment of potential Scottish blue carbon stores by Burrows et al. (2014). In particular, the total stock of surficial sediments has been revised from ~2,330 Mt C (Burrows et al., 2014) to ~2,600 Mt C (Smeaton et al., 2021a). In addition to the blue carbon habitats reported in Burrows et al. (2014), machair, sand dunes and bryozoan thickets have also been included in this review. Extent estimates for various habitats, namely seagrass and maerl, have also been revised using data from GeMS. In total, Burrows et al. (2014) estimated ~1 Mt C to be stored within Scottish habitats, with this current review reporting an increase to ~5.4 Mt C (3.1 Mt OC; 2.3 Mt IC). This change comes from the inclusion of additional habitats and improved extent and area-specific carbon stock estimates.
6.8 Carbon emissions
Using organic carbon only, at least 68,000 t CO2-eq are sequestered annually by coastal and nearshore blue carbon habitats in the Scottish coastal and marine environment, with a further 308,280 t CO2-eq buried annually within fjordic sediments (Table 5). Combined, this is an estimated annual sequestration rate of at least 0.4 Mt CO2-eq yr-1. A previous estimate of 28.4 Mt CO2-eq yr-1 reported by Burrows et al., (2014) was significantly higher because it included seabed sediments. We have chosen not to estimate a sequestration rate for the large area of shelf seabed sediments due to limited constraining data. However, we acknowledge that the estimated annual rate of 0.4 Mt CO2-eq yr-1 reported above, will be significantly lower than reality. For context, Scottish Greenhouse Gas emissions (GHG) for 2020 were 40 Mt CO2e (Scottish Government, 2023). Thus, the CO2 sequestered annually through Scottish blue carbon that we can currently account for represents approximately 1% of these emissions.
Table 5. Summary of the total storage and sequestration capacity for Scottish blue carbon habitats converted to approximate values of CO2 emissions where OC stocks have been calculated. To provide CO2 equivalents, values for carbon are multiplied by the IPCC conversion factor, 3.67 (molecular mass/atomic mass). UP indicates an unknown or inapplicable parameter.
BC Habitat | Total standing stock (t CO2-eq) | Sequestration capacity (t CO2-eq yr-1) |
---|---|---|
Machair |
1,500,000 |
15,000 |
Sand dunes |
2,763,341 |
33,078 |
Saltmarsh |
4,127,861 |
16,093 |
Seagrass beds |
607,165 |
3,747 |
Kelp beds |
2,202,000 |
5,198,000 [a] |
Maerl [b] |
UP |
UP |
Cold-water coral reefs [b] |
UP |
UP |
Serpulid aggregations [b] |
UP |
UP |
Flame shell [b] |
UP |
UP |
Horse mussel beds [b] |
UP |
UP |
Blue mussel beds [b] |
UP |
UP |
Native oyster [b] |
UP |
UP |
Brittlestar [b] |
UP |
UP |
Bryozoan communities [b] |
UP |
UP |
Total |
11.3 x 106 |
67,877 [c] |
Sediment |
1,308.4 x 106 [d] |
308,280 [e] |
Total |
1,319.6 x 106 |
376,157 |
[a] Kelp does not sequester this locally – this value should be considered as the production capacity.
[b] Due to the nature of carbonate chemistry and recalcitrant property of carbonate material (Frankignoulle and Gattuso, 1994), it is highly unlikely that inorganic carbon stores will result in CO2 emissions. The IC standing stock values have therefore not been converted to a CO2-equivalent value. It is not currently known what the net sequestration capacity for calcifying aggregations is; carbonate production is a net source of CO2, however photosynthesis (e.g., by maerl) and burial of OC within and below reef structures may constitute a sink.
[c]Does not include kelp production capacity or carbonate production capacities.
[d] The value is based on the total carbon stored in the top 10 cm of sediments within the Scottish EEZ; only the organic component (14%) is potentially susceptible to remineralisation to CO2.
[e] This is based on the burial rate of Sottish fjords, ~84,000 t C yr-1 (Smeaton et al., 2021b).
This review estimates that Scottish coastal and nearshore blue carbon habitats have an existing standing stock of 11.3 Mt CO2-eq, including OC contributions only. This figure is comparable to the GHG emissions released in 2020 by the largest Scottish sector, transportation - excluding international aviation and shipping - totalling 9.5 Mt CO2-eq (Scottish Government, 2023). A further estimated 1,310 Mt CO2-eq are held within the organic fraction of surficial sediments across the Scottish portion of the UK EEZ, which is an amount equivalent to over 30 years’ worth of Scotland’s 2020 GHG emissions. However, the proportion of OC potentially vulnerable to remineralisation is uncertain due to complex biogeochemical factors, and it is likely that this will be limited to the upper sediment layers that experience more frequent disturbance, and the less refractory material (Smeaton et al., 2021a; Smeaton et al., 2022a; Black et al., 2022). Research is ongoing within this area (Sala et al., 2021; Hiddink et al., 2023)
Conclusions
A larger focus has traditionally been given to terrestrial systems when considering carbon sequestration and stocks. However, this review highlights the important role that Scottish coastal and marine habitats play in carbon sequestration and in mitigating the impacts of climate change. There are however still large evidence gaps and uncertainties for most of the habitats and values presented here. Initial assessments of data confidence have been made during this review and key evidence gaps identified, to help in prioritisation of future work.
Whilst their contribution to carbon sequestration and stocks is significant, it is important to maintain a holistic approach when considering blue carbon habitats. Blue carbon is only one element of a range of ecosystem services provided by these coastal and marine habitats. The connectivity and inter-relationships between blue carbon habitats is also an important factor, recognising the highly dynamic nature of marine and estuarine systems. .
Addressing key evidence gaps will support the development of evidence-based policies for effective protection, restoration and management of our blue carbon assets, to secure the health of these habitats and the wider ecosystem and societal benefits they can provide.
References
Adams, C.A., Andrews, J.E. and Jickells, T. 2012. Nitrous oxide and methane fluxes vs. carbon, nitrogen and phosphorous burial in new intertidal and saltmarsh sediments. Science of the Total Environment, 434, 240-251.
Adaptation Scotland, 2022. Climate trends and projections.
Adnitt C., Brew, D., Cottle, R., Hardwick, M., John, S., Leggett, D., McNulty, S., Meakins, N. and Staniland, R., 2007. Saltmarsh management manual. Environment Agency, Bristol.
Aitkenhead M, Coull M, 2019. Mapping soil profile depth, bulk density and carbon stock in Scotland using remote sensing and spatial covariates. European Journal of Soil Science, 71, 553–567.
Allen, C., Axelsson, M., Dewey, S. and Wilson, J. 2014. Fal and Helford SAC maerl dropdown video and dive survey 2013. A report to Natural England by Seastar Survey Ltd.
Aller, R.C. 1982. Carbonate dissolution in nearshore terrigenous muds: the role of physical and biological reworking. The Journal of Geology, 90, 79-95.
Angus, S. and Hansom, J. 2004. Tir a'Mhachair, Tir nan Loch? Climate change scenarios for Scottish machair systems: a wetter future? Delivering Sustainable Coasts: Connecting Science and Policy.
Angus, S. and Hansom, J.D. 2021. Enhancing the resilience of high-vulnerability, low-elevation coastal zones. Ocean & Coastal Management, 200(2), 105414.
Angus, S. and Rennie, A. 2014. An Ataireachd Aird: The storm of January 2005 in the Uists, Scotland. Ocean & Coastal Management, 94, 22-29.
Angus, S. 2001. The Outer Hebrides, Moor and Machair. The White Horse Press, Cambridge & Harris, 339 pp.
Angus, S. 2018. Beyond the meta-ecosystem? The need for a multi-faceted approach to climate change in low-lying coastal wetlands: the case of South Uist, Scotland. Ocean & Coastal Management, 165, 334-345.
Angus, S., Hansom, J.D. and Rennie, A. 2011. Habitat Change on Scotland’s Coasts – The Changing Nature of Scotland. In: Marrs, S.J., Foster, S., Hendrie, C., Mackey, E.C., and Thompson, D.B.A. (eds). TSO Scotland, Edinburgh, pp.183-198.
Armstrong, S., Hull, S., Pearson, Z., Wilson, R. and Kay, S. 2020. Estimating the Carbon Sink Potential of the Welsh Marine Environment. NRW, Cardiff, p.74.
Aronson, R.B. 1989. Brittlestar beds: low-predation anachronisms in the British Isles. Ecology, 70, 856-865.
Arzul, I., Gagnaire, B., Bond, C., Chollet, B., Morga, B., Ferrand, S., Robert, M. and Renault, T. 2009. Effects of temperature and salinity on the survival of Bonamia ostreae, a parasite infecting flat oysters Ostrea edulis. Diseases of aquatic organisms, 85(1), 67-75.
Atwood, T.B., Witt, A.W., Mayorga, J., Hammill, E. and Sala, E. 2020. Global patterns in marine sediment carbon stocks. Frontiers in Marine Science, 7(165), 1-9.
Austin, W.E.N., Smeaton, C., Riegel, S., Ruranska, P. and Miller, L. 2021. Blue carbon stock in Scottish saltmarsh soils. Scottish Marine and Freshwater Science, 12(13), 37.
Austin, W., Smeaton, C., Houston, A. and Balke, T. 2022. Scottish saltmarsh, sea-level rise, and the potential for managed realignment to deliver blue carbon gains. ClimateXChange Technical Report. Edinburgh, UK.
Baden, S., Hernroth, B. and Lindahl, O. 2021. Declining Populations of Mytilus spp. in North Atlantic Coastal Waters—A Swedish Perspective. Journal of Shellfish Research, 40(2), 269-296.
Bartholomeus, R., Witte, J. and Runhaar, J. 2011. Drought stress and vegetation characteristics on sites with different slopes and orientations. Ecohydrology, 5(6), 808-818.
Bauer, J.E., Cai, W.J., Raymond, P.A., Bianchi, T.S., Hopkinson, C.S. and Regnier, P.A. 2013. Global patterns in marine sediment carbon stocks. Nature, 504(7478), 61-70.
Beaumont, N., Jones, L., Garbutt, A., Hansom, J. and Toberman, M., 2014. The value of carbon sequestration and storage in coastal habitats. Estuarine, Coastal and Shelf Science, 137, 32-40.
Begg, T., Graham, J. and Matejusova, I. 2020. The Marine Invasive Non-Native Species Didemnum vexillum: Loch Creran Survey – September 2019. Scottish Marine and Freshwater Science, 11(5), 17.
Bianchi, T.S., 2011. The role of terrestrially derived organic carbon in the coastal ocean: A changing paradigm and the priming effect. Proceedings of the National Academy of Sciences, 108(49), 19473-19481.
Black, K.E., Smeaton, C., Turrell, W.R. and Austin, W.E.N. 2022. Assessing the potential vulnerability of sedimentary carbon stores to bottom trawling disturbance within the UK EEZ. Frontiers in Marine Science, 9:892892.
Bosence, D. and Wilson, J. 2003. Maerl growth, carbonate production rates and accumulation rates in the NE Atlantic. Aquatic Conservation: Marine and Freshwater Ecosystems, 13(S1), S21-S31.
Bradshaw, C., Veale, L.O., Hill, A.S. and Brand, A.R. 2002. The role of scallop-dredge disturbance in long-term changes in Irish Sea benthic communities: a re-analysis of an historical dataset. Journal of Sea Research, 47, 161-184.
Brown, R.A. 1984. Geographical variations in the reproduction of the horse mussel, Modiolus modiolus (Mollusca: Bivalvia). Journal of the Marine Biological Association of the United Kingdom, 64(4), 751-770.
Burden, A., Garbutt, R.A., Evans, C.D., Jones, D. and Cooper, D. 2013. Carbon sequestration and biogeochemical cycling in a saltmarsh Subject to coastal managed realignment. Estuarine, Coastal and Shelf Science, 120, 12-20.
Burden, A., Smeaton, C., Angus, S., Garbutt, A., Jones, L., Lewis H.D. and Rees. S.M. 2020. Impacts of climate change on coastal habitats relevant to the coastal and marine environment around the UK. MCCIP Science Review 2020, 228-255.
Burrows, M.T., Twigg, G., Mieszkowska, N. and Harvey, R. 2017. Marine Biodiversity and Climate Change (MarClim): Scotland 2014/15. Scottish Natural Heritage Commissioned Report No. 939.
Burrows M.T., Fox, C.J., Moore, P., Smale, D., Sotheran, I., Benson, A., Greenhill, L., Martino, S., Parker, A., Thompson, E. and Allen, C.J. 2018. Wild Seaweed Harvesting as a Diversification Opportunity for Fishermen. A report by SRSL for HIE, p.171.
Burrows M.T., Kamenos N.A., Hughes D.J., Stahl H., Howe J.A. and Tett P. 2014. Assessment of carbon budgets and potential blue carbon stores in Scotland’s coastal and marine environment. Scottish Natural Heritage Commissioned Report No. 761.
Burrows, M.T., Hughes, D.J., Austin, W.E.N., Smeaton, C., Hicks, N., Howe, J.A., Allen, C.,Taylor, P. and Vare, L.L. 2017. Assessment of Blue Carbon Resources in Scotland’s Inshore Marine Protected Area Network. Scottish Natural Heritage Commissioned Report No. 957.
Büscher, J.V., Form, A.U. and Riebesell, U. 2017. Interactive effects of ocean acidification and warming on growth, fitness and survival of the cold-water coral Lophelia pertusa under different food availabilities. Frontiers in Marine Science, 4, 101.
Cannell, M.G.R., Milne, R., Hargreaves, K.J., Brown, T.A.W., Cruickshank, M.M., Bradley, R.I., Spencer, T., Hope, D., Billett, M.F., Adger, W.N. and Subak, S. 1999. National inventories of terrestrial carbon sources and sinks: the UK experience. Climatic Change, 42(3), 505-530.
Chambers, P.M., Blampied, S., Binney, F., Austin, W.E.N., and Morel, G. 2022a. Blue carbon resources: an assessment of Jersey’s territorial seas. Government of Jersey.
Chambers, C., Jones, K.L., Malcolm, F., Boyle, G., Sanderson, W.G., Garbutt, A., Jones, L., Unsworth, R.K.F. and Lilley, R.J. 2022b. Development of a marine and coastal enhancement project assessment framework for Scottish inshore waters. NatureScot Research Report No. 1293.
Chapman, S.J., Bell, J., Donnelly, D. and Lilly, A. 2009. Carbon stocks in Scottish peatlands. Soil Use and Management, 25(2), 105-112.
Chmura, G.L., Anisfeld, S.C., Cahoon, D.R. and Lynch, J.C. 2003. Global carbon sequestration in tidal, saline wetland soils. Global Biogeochemical Cycles, 17(1111).
Climate Change Committee, 2022. Briefing: Blue Carbon.
Collin, S.B., Tweddle, J.F. and Shucksmith, R.J. 2015. Rapid assessment of marine non-native species in the Shetland Islands, Scotland. BioInvasions Records, 4(3), 147-155.
Collins, M.J. 1986. Taphonomic processes in a deep water Modiolus-brachiopod assemblage from the west coast of Scotland. PhD thesis, University of Glasgow.
Cui, X., Bianchi, T.S., Savage, C. and Smith, R.W. 2016. Organic carbon burial in fjords: Terrestrial versus marine inputs. Earth and Planetary Science Letters, 451, 41-50.
d’Avack, E. A. S., Tillin, H. M., Jackson, E. L. and Tyler-Walters, H. 2014. Assessing the sensitivity of seagrass bed biotopes to pressures associated with marine activities. JNCC Report no. 505.
Dahl, M., Asplund, M.E., Deyanova, D., Franco, J.N., Koliji, A., Infantes, E., Perry, D., Björk, M. and Gullström, M. 2020. High seasonal variability in sediment carbon stocks of cold‐temperate seagrass meadows. Journal of Geophysical Research: Biogeosciences, 125(1).
Dargie, T.C.D. 2000. Sand Dune Vegetation Survey of Scotland: National Report. Volume 1. Scottish Natural Heritage.
Davidson, K.E., Fowler, M.S., Skov, M.W., Doerr, S.H., Beaumont, N. and Griffin, J.N. 2017. Livestock grazing alters multiple ecosystem properties and services in salt marshes: A meta‐analysis. Journal of Applied Ecology, 54(5), 1395-1405.
Davies, A.J., Green, S.L., Long, D. and Roberts, J.M. 2009. Developing the necessary data layers to inform the development of a site boundary for the East Mingulay dSAC – Phase II. Scottish Natural Heritage Commissioned Report No. 306.
De Clippele, L.H., Rovelli, L., Ramiro-Sánchez, B., Kazanidis, G., Vad, J., Turner, S., Glud, R.N. and Roberts, J.M. 2021. Mapping cold-water coral biomass: an approach to derive ecosystem functions. Coral Reefs, 40, 215-231.
De Santo, E.M. 2013. The Darwin Mounds special area of conservation: Implications for offshore marine governance. Marine Policy, 41, 25-32.
De Santo, E.M. and Jones, P.J. 2007. The Darwin Mounds: from undiscovered coral to the development of an offshore marine protected area regime. Bulletin of Marine Science, 81(3), 147-156.
De-Bastos, E.S.R. and Hill, J. 2016. Ophiothrix fragilis and/or Ophiocomina nigra brittlestar beds on sublittoral mixed sediment. In: Tyler-Walters, H. and Hiscock, K. (eds). Marine Life Information Network: Biology and Sensitivity Key Information Reviews. Plymouth, Marine Biological Association of the United Kingdom.
Department for Business, Energy and Industrial Strategy (DBEIS), 2011. A brief guide to the carbon valuation methodology for UK policy appraisal, October, 2011.
Diesing, M., Kröger, S., Parker, R., Jenkins, C., Mason, C. and Weston, K. 2017. Predicting the standing stock of organic carbon in surface sediments of the North–West European continental shelf. Biogeochemistry, 135(1-2), 183-200.
Dodd, J., Baxter, L. and Hughes, D.J. 2009. Mapping Serpula vermicularis (Polychaeta: Serpulidae) aggregations in Loch Teacuis, western Scotland, a new record. Marine Biology Research, 5(2), 200-205.
Doney, S.C., Fabry, V.J., Feely, R.A. and Kleypas, J.A. 2009. Ocean acidification: the other CO2 problem. Annual Review of Marine Science, 1, 169-192.
Douarin, M., Sinclair, D.J., Elliot, M., Henry, L.A., Long, D., Mitchison, F. and Roberts, J.M. 2014. Changes in fossil assemblage in sediment cores from Mingulay Reef Complex (NE Atlantic): Implications for coral reef build-up. Deep Sea Research Part II: Topical Studies in Oceanography, 99, 286-296.
Duarte, C., Marbà, N., Gacia, E., Fourqurean, J., Beggins, J., Barrón, C. and Apostolaki, E. 2010. Seagrass community metabolism: Assessing the carbon sink capacity of seagrass meadows. Global Biogeochemical Cycles, 24(4).
Duarte, C., Middelburg, J. and Caraco, N. 2005. Major role of marine vegetation on the oceanic carbon cycle. Biogeosciences, 2(1), 1-8.
Duarte, C.M., Agusti, S., Barbier, E., Britten, G.L., Castilla, J.C., Gattuso, J.P., Fulweiler, R.W., Hughes, T.P., Knowlton, N., Lovelock, C.E. and Lotze, H.K. 2020. Rebuilding marine life. Nature, 580(7801), 39-51.
Duarte, C. M., Bruhn, A. and Krause-Jensen, D. 2021. A seaweed aquaculture imperative to meet global sustainability targets. Nature Sustainability, 1-9.
Dupont, S., Havenhand, J., Thorndyke, W., Peck, L. and Thorndyke, M. 2008. Near-future level of CO2-driven ocean acidification radically affects larval survival and development in the brittlestar Ophiothrix fragilis. Marine Ecology Progress Series, 373, 285-294.
Dynamic Coast, 2023. Dynamic Coast: About.
Eigaard, O.R., Bastardie, F., Breen, M., Dinesen, G.E., Hintzen, N.T., Laffargue, P., et al. 2016. Estimating seabed pressure from demersal trawls, seines, and dredges based on gear design and dimensions. ICES Journal of Marine Science, 73(9), 2420-242
Elith, J., Phillips, S.J., Hastie, T., Dudík, M., Chee, Y.E. and Yates, C.J. 2011. A statistical explanation of MaxEnt for ecologists. Diversity and distributions, 17(1), 43-57.
Elliott, S. 2015. Coastal realignment at RSPB Nigg Bay nature reserve. RSPB Internal Report.
Elschot, K., Bakker, J.P., Temmerman, S., van de Koppel, J. and Bouma, T.J. 2015. Ecosystem engineering by large grazers enhances carbon stocks in a tidal salt marsh. Marine Ecology Progress Series, 537, 9-21.
Elschot, K., Bouma, T.J., Temmerman, S. and Bakker, J.P. 2013. Effects of long-term grazing on sediment deposition and salt-marsh accretion rates. Estuarine, Coastal and Shelf Science, 133, 109-115.
Engelsma, M.Y., Kerkhoff, S., Roozenburg, I., Haenen, O.L., Van Gool, A., Sistermans, W., Wijnhoven, S. and Hummel, H. 2010. Epidemiology of Bonamia ostreae infecting European flat oysters Ostrea edulis from Lake Grevelingen, The Netherlands. Marine Ecology Progress Series, 409, 131-142.
Evans, C., Artz, R., Moxley, J., Smyth, M.A., Taylor, E., Archer, E., Burden, A., Williamson, J., Donnelly, D., Thomson, A., Buys, G., Malcolm, H., Wilson, D. and Renou-Wilson, F. 2017. Implementation of an emissions inventory for UK peatlands. Report to the Department for Business, Energy and Industrial Strategy. Centre for Ecology and Hydrology, Bangor, 88pp.
Everard, M., Jones, L. and Watts, B. 2010. Have we neglected the societal importance of sand dunes? An ecosystem services perspective. Aquatic conservation marine and freshwater ecosystems, 20, 476-487.
Fagherazzi, S., Mariotti, G., Leonardi, N., Canestrelli, A., Nardin, W. and Kearney, W.S. 2020. Salt Marsh Dynamics in a Period of Accelerated Sea Level Rise. Journal of Geophysical Research: Earth Surface, 125(8).
Fariñas-Franco, J.M., Pearce, B., Mair, J.M., Harries, D.B., MacPherson, R.C., Porter, J.S., Reimer, P.J. and Sanderson, W.G. 2018. Missing native oyster (Ostrea edulis) beds in a European Marine Protected Area: Should there be widespread restorative management? Biological Conservation, 221, 293-311.
FeAST, 2013. Feature Activity Sensitivity Tool.
Fernández, C. 2011. The retreat of large brown seaweeds on the north coast of Spain: the case of Saccorhiza polyschides. European Journal of Phycology, 46(4), 352-360.
Ferretto, A., Brooker, R., Aitkenhead, M., Matthews, R. and Smith, P. 2019. Potential carbon loss from Scottish peatlands under climate change. Regional Environmental Change, 19(7), 2101-2111.
Filbee-Dexter, K., Wernberg, T., Norderhaug, K.M., Ramirez-Llodra, E. and Pedersen, M.F. 2018. Movement of pulsed resource subsidies from kelp forests to deep fjords. Oecologia, 187(1), 291-304.
Filbee-Dexter, K. and Wernberg, T. 2020. Substantial blue carbon in overlooked Australian kelp forests. Scientific Reports, 10(1).
Filbee-Dexter, K., Feehan, C., Smale, D., Krumhansl, K., Augustine, S., de Bettignies, F., Burrows, M.T., Byrnes, J., Campbell, J., Davoult, D. and Dunton, K. 2020. Ocean temperature controls kelp decomposition and carbon sink potential.
Fitzer, S.C., Vittert, L., Bowman, A., Kamenos, N.A., Phoenix, V.R. and Cusack, M. 2015. Ocean acidification and temperature increase impact mussel shell shape and thickness: problematic for protection? Ecology and evolution, 5(21), 4875-4884.
Ford, H., Garbutt, A., Jones, D.L. and Jones, L. 2012. Impacts of grazing abandonment on ecosystem service provision: coastal grassland as a model system. Agriculture, Ecosystems & Environment, 162, 108-115.
Forestry Commission, 2022. Forestry Statistics, 2022. Chapter 4: Carbon. Forest Research.
Fourqurean, J., Duarte, C., Kennedy, H., Marbà, N., Holmer, M., Mateo, M., Apostolaki, E., Kendrick, G., Krause-Jensen, D., McGlathery, K. and Serrano, O. 2012. Seagrass ecosystems as a globally significant carbon stock. Nature Geoscience, 5(7), 505-509.
Frankignoulle, M., Canon, C. and Gattuso, J.P. 1994 Marine calcification as a source of carbon dioxide: Positive feedback of increasing atmospheric CO2. Limnology and Oceanography, 39, 458-462.
Freiwald, A. and Henrich, R. 1994. Reefal coralline algal build‐ups within the Arctic Circle: morphology and sedimentary dynamics under extreme environmental seasonality. Sedimentology, 41(5), 963-984.
Galy, V., Peucker-Ehrenbrink, B. and Eglinton, T. 2015. Global carbon export from the terrestrial biosphere controlled by erosion. Nature, 521(7551), 204-207.
Garrard, S.M., Perry, F. and Tyler-Walters, H. 2019. Lophelia reefs. In: Tyler-Walters, H. and Hiscock, K. (eds). Marine Life Information Network: Biology and Sensitivity Key Information Reviews. Plymouth: Marine Biological Association of the United Kingdom.
Gass, S.E. and Roberts, J.M. 2006. The occurrence of the cold-water coral Lophelia pertusa (Scleractinia) on oil and gas platforms in the North Sea: colony growth, recruitment and environmental controls on distribution. Marine Pollution Bulletin, 52(5), 549-559.
Govers, L.L., Heusinkveld, J.H.T., Gräfnings, M.L.E., Smeele, Q. and van der Heide, T. 2022. Adaptive intertidal seed-based seagrass restoration in the Dutch Wadden Sea. PLoS ONE 17(2): e0262845.
Glud, R.N. 2008. Oxygen dynamics of marine sediments. Marine Biology Research, 4(4), 243-289.
Gormley, K.S., Porter, J.S., Bell, M.C., Hull, A.D. and Sanderson, W.G. 2013. Predictive habitat modelling as a tool to assess the change in distribution and extent of an OSPAR priority habitat under an increased ocean temperature scenario: consequences for marine protected area networks and management. PLoS One, 8(7).
Grech, A., Coles, R. and Marsh, H. 2011. A broad-scale assessment of the risk to coastal seagrasses from cumulative threats. Marine Policy, 35(5), 560-567.
Green, A., Chadwick, M. and Jones, P. 2018. Variability of UK seagrass sediment carbon: Implications for blue carbon estimates and marine conservation management. PLoS One, 13(9).
Green A.E., Unsworth, R.K.F., Chadwick, M.A. and Jones P.J.S. 2021. Historical Analysis Exposes Catastrophic Seagrass Loss for the United Kingdom. Frontiers in Plant Science, 12.
Green, E.E.P. and Short, F.T. 2003. World atlas of seagrasses. University of California, Princeton.
Gregg, R., Elias, J.L., Alonso, I.E., Muto, P. and Morecroft, M.D. 2021. Carbon storage and sequestration by habitat: a review of the evidence (second edition). Natural England Research Report NERR094. York.
Greiner, J.T., McGlathery, K.J., Gunnell, J. and McKee, B.A. 2013. Seagrass restoration enhances “blue carbon” sequestration in coastal waters. PLoS One, 8(8).
Hall-Spencer, J.M. and Moore, P.G. 2000a. Scallop dredging has profound, long-term impacts on maerl habitats. ICES Journal of Marine Science, 57(5), 1407-1415.
Hall-Spencer, J.M. and Moore, P.G. 2000b. Limaria hians (Mollusca: Limacea): a neglected reef-forming keystone species. Aquatic Conservation: Marine and Freshwater Ecosystems, 10, 267-277.
Hall-Spencer, J.M. and Stehfest, K.M. 2009. Background Document for Lophelia pertusa reefs. Joint Nature Conservation Committee, Peterborough, UK.
Hall-Spencer, J.M., Kelly, J. and Maggs, C.A. 2008. Assessment of maerl beds in the OSPAR area and the development of a monitoring program. Department of Environment, Heritage and Local Government: Ireland.
Hall-Spencer, J., White, N., Gillespie, E., Gillham, K. and Foggo, A. 2006. Impact of fish farms on maerl beds in strongly tidal areas. Marine Ecology Progress Series, 326, 1-9.
Hansom, J., Maxwell, F., Naylor, L. and Piedra, M. 2017. Impacts of sea-level rise and storm surges due to climate change in the Firth of Clyde. Scottish Natural Heritage Commissioned Report No. 891.
Hansom, J.D. and McGlashan, D.J. 2004. Scotland's coast: Understanding past and present processes for sustainable management. Scottish Geographical Journal, 120.
Hansom, J.D., Lees, R.G., Maslen, J., Tilbrook, C. and McManus, J. 2001. Coastal dynamics and sustainable management: the potential for managed realignment in the Forth estuary. In: Gordon, J.E. and Lees, K.F. (eds). Earth Science and the Natural Heritage. Edinburgh: The Stationery Office, pp.148-160.
Harvey, R.J., Garbutt, A., Hawkins, S.J. and Skov, M.W. 2019. No detectable broad-scale effect of livestock grazing on soil blue-carbon stock in salt marshes. Frontiers in Ecology and Evolution, 7, 151.
Haskoning UK Ltd., 2006. Investigation into the impact of marine fish farm deposition on maerl beds. Scottish Natural Heritage Commissioned Report No. 213
Haynes, T.A. 2016. Scottish saltmarsh survey national report. Scottish Natural Heritage Commissioned Report, No. 786.
Hedges, J.I., Keil, R.G. and Benner, R. 1997. What happens to terrestrial organic matter in the ocean? Organic geochemistry, 27(5-6), 195-212.
Hennige, S.J., Wolfram, U., Wickes, L., Murray, F., Roberts, J.M., Kamenos, N.A., Schofield, S., Groetsch, A., Spiesz, E.M., Aubin-Tam, M.E. and Etnoyer, P.J. 2020. Crumbling Reefs and Cold-Water Coral Habitat Loss in a Future Ocean: Evidence of “Coralporosis” as an Indicator of Habitat Integrity. Frontiers in Marine Science, 7, 668.
Hiddink, J.G., van de Velde, S.J., McConnaughey, R.A., De Borger, E., Tiano, J., Kaiser, M.J., Sweetman, A.K. and Sciberras, M. 2023. Quantifying the carbon benefits of ending bottom trawling. Nature, 617(7960), E1-E2.
Hill, M.O. and Wallace, H.L. 1989. Vegetation and environment in afforested sand dunes at Newborough, Anglesey. Forestry: An International Journal of Forest Research, 62(3), 249-267.
Hily, C., Grall, J., Chauvaud, L., Lejart, M. and Clavier, J. 2013. CO2 generation by calcified invertebrates along rocky shores of Brittany, France. Marine and freshwater research, 64, 91-101.
Hirst, N.E., Clark, L. and Sanderson, W.G. 2012. The distribution of selected MPA search features and Priority Marine Features off the NE coast of Scotland. Scottish Natural Heritage Commissioned Report, No. 500.
Hiscock, K., Sewell, J. and Oakley, J. 2005. Marine health check 2005: A report to gauge the health of the UK's sea-life. WWF-UK.
Holt, T., Rees, E. I.S. and Hawkins, S.J. 1998. Biogenic reefs (Volume IX). An overview of dynamic and sensitivity characteristics for conservation management of marine SACs. Scottish Association for Marine Science (UK Marine SACs Project), p.170.
Hootsmans, M.J.M., Vermaat, J.E. and Van Vierssen, W. 1987. Seed-bank development, germination and early seedling survival of two seagrass species from The Netherlands: Zostera marina L. and Zostera noltii hornem. Aquatic Botany, 28(3-4), 275-285.
Horton, B.P., Shennan, I., Bradley, S.L., Cahill, N., Kirwan, M., Kopp, R.E. and Shaw, T.A. 2018. Predicting marsh vulnerability to sea-level rise using Holocene relative sea-level data. Nature communications, 9(1), 1-7.
Hourigan, T.F. 2009. Managing fishery impacts on deep-water coral ecosystems of the USA: emerging best practices. Marine Ecology Progress Series, 397, 333-340.
Hughes, D.J. 1998. Subtidal brittlestar beds (volume IV). An Overview of Dynamics and Sensitivity Characteristics for Conservation Management of Marine SACs. Scottish Association for Marine Science (UK Marine SACs Project), p.78.
Hughes, D.J., Poloczanska, E.S. and Dodd, J. 2008. Survivorship and tube growth of reef‐building Serpula vermicularis (Polychaeta: Serpulidae) in two Scottish sea lochs. Aquatic Conservation: marine and freshwater ecosystems, 18(2), 117-129.
Hunt, C., Demšar, U., Dove, D., Smeaton, C., Cooper, R. an Austin W.E.N. 2020. Quantifying Marine Sedimentary Carbon: A New Spatial Analysis Approach Using Seafloor Acoustics, Imagery, and Ground-Truthing Data in Scotland. Frontiers in Marine Science, 7.
Hunt, C., Demšar, U., Marchant, B., Dove, D., Austin W.E.N. 2021. Sounding Out the Carbon: The Potential of Acoustic Backscatter Data to Yield Improved Spatial Predictions of Organic Carbon in Marine Sediments. Frontiers in Marine Science, 8.
Hutchison, Z.L., Hendrick, V.J., Burrows, M.T., Wilson, B. and Last, K.S. 2016. Buried alive: the behavioural response of the mussels, Modiolus modiolus and Mytilus edulis to sudden burial by sediment. PLoS One, 11(3).
Huvenne, V.A.I., Bett, B.J., Masson, D.G., Le Bas, T.P. and Wheeler, A.J. 2016. Effectiveness of a deep-sea cold-water coral Marine Protected Area, following eight years of fisheries closure. Biological Conservation, 200, 60-69.
IPCC, 2014. Climate Change 2014: Synthesis Report. Contribution of Working Groups I, II and III to the Fifth Assessment Report of the Intergovernmental Panel on Climate Change [Core Writing Team, R.K. Pachauri and L.A. Meyer (eds.)]. IPCC, Geneva, Switzerland, 151 pp.
IPCC, 2019. Climate Change and Land: an IPCC special report on climate change, desertification, land degradation, sustainable land management, food security, and greenhouse gas fluxes in terrestrial ecosystems. In: Shukla, P.R., Skea, J., Calvo Buendia, E., Masson-Delmotte, V., Pörtner, H.-O., Roberts, D.C., Zhai, P., Slade, R., Connors, S., van Diemen, R., Ferrat, M., Haughey, E., Luz, S., Neogi, S., Pathak, M., Petzold, J., Portugal Pereira, J., Vyas, P., Huntley, E., Kissick, K., Belkacemi, M., Malley, J. (eds).
Jackson, E.L., Davies, A.J., Howell, K.L., Kershaw, P.J. and Hall-Spencer, J.M. 2014. Future-proofing marine protected area networks for cold water coral reefs, ICES Journal of Marine Science, 71(9), 2621–2629.
Jansen, H. and van den Bogaart, L. 2020. Blue carbon by marine bivalves. Perspective of Carbon sequestration by cultured and wild bivalve stocks in the Dutch coastal areas, s.l.: Wageningen Marine Research.
Jennings, S. and Kaiser, M.J. 1998. The effects of fishing on marine ecosystems. Advances in Marine Biology, 34, 201-352.
JNCC, 2007. Second Report by the UK under Article 17 on the implementation of the Habitats Directive from January 2001 to December 2006. JNCC, Peterborough.
JNCC, 2021. West Sands Dune Management. Nature-based Solutions: IACCG case studies.
Jones, M.L.M., Angus, S., Cooper, A., Doody, P., Everard, M., Garbutt, A., Gilchrist, P., Hansom, G, Nicholls, R., Pye, K., Ravenscroft, N., Rees, S., Rhind, P. and Whitehouse, A. 2011. Coastal margins [Chapter 11]. In: UK National Ecosystem Assessment. Understanding nature’s value to society. UNEPWCMC, Cambridge, pp.411-457.
Jones, M.L.M., Sowerby, A., Williams, D.L. and Jones, R.E. 2008. Factors controlling soil development in sand dunes: evidence from a coastal dune soil chronosequence. Plant Soil, 307(1-2), 219-234.
Jones, M.L.M., Wallace, H.L., Norris, D., Brittain, S.A., Haria, S., Jones, R.E., Rhind, P.M., Reynolds, B.R. and Emmett, B.A. 2004. Changes in vegetation and soil characteristics in coastal sand dunes along a gradient of atmospheric nitrogen deposition. Plant Biology, 6(5), 598-605.
Kain, J.M. 1979. A view of the genus Laminaria. Oceanography and Marine Biology an Annual Review, 17, 101-161.
Kamenos, N.A., Burdett, H.L., Aloisio, E., Findlay, H.S., Martin, S., Longbone, C., Dunn, J., Widdicombe, S. and Calosi, P. 2013. Coralline algal structure is more sensitive to rate, rather than the magnitude, of ocean acidification. Global Change Biology, 19(12), 3621-3628.
Kamphausen, L., Epstein, G., Henderson, S., Steel, L., MacMinn, R., Dodd, J. and James, B. 2015. Loch Teacuis serpulid survey March 2015. Scottish Natural Heritage post-survey summary.
Kaskela, A., Kotilainen, A., Alanen, U., Cooper, R., Green, S., Guinan, J., van Heteren, S., Kihlman, S., Van Lancker, V. and Stevenson, A. 2019. Picking Up the Pieces—Harmonising and Collating Seabed Substrate Data for European Maritime Areas. Geosciences, 9(2), 84.
Kennedy, H., Beggins, J., Duarte, C., Fourqurean, J., Holmer, M., Marbà, N. and Middelburg, J. 2010. Seagrass sediments as a global carbon sink: Isotopic constraints. Global Biogeochemical Cycles, 24(4).
Kennon, N.A., Robertson-Jones, A., Jemmett, S., Hugh-Jones, T., Bell, M.C. and Sanderson, W.G. 2023. Rotational fishing enables biodiversity recovery and provides a model for oyster (Ostrea edulis) habitat restoration. PLOS ONE 18(3): e0283345.
Kent, F.E.A., Gray, M.J., Last, K.S. and Sanderson, W.G. 2016. Horse mussel reef ecosystem services: evidence for a whelk nursery habitat supporting a shellfishery, International Journal of Biodiversity Science, Ecosystem Services & Management, 12(3), 172-180.
Kent, F., Lilley, R., Unsworth, R., Cunningham, S., Begg, T., Boulcott, P., Jeorrett, C., Horsburgh, R. and Michelotti, M. 2021. Seagrass restoration in Scotland - handbook and guidance. NatureScot Research Report 1286.
King, N.G., Moore, P.J., Pessarrodona, A., Burrows, M.T., Porter, J., Bue, M. and Smale, D.A. 2020. Ecological performance differs between range centre and trailing edge populations of a cold-water kelp: implications for estimating net primary productivity. Marine Biology, 167(9).
Kingham, R. 2013. The broad-scale impacts of livestock grazing on saltmarsh carbon stocks. Doctoral dissertation, Bangor University.
Krause-Jensen, D. and Duarte, C. 2016. Substantial role of macroalgae in marine carbon sequestration Nature Geoscience, 9(10), 737-742.
Krause-Jensen, D., Lavery, P., Serrano, O., Marbà, N., Masque, P. and Duarte, C.M. 2018. Sequestration of macroalgal carbon: the elephant in the Blue Carbon room. Biology letters, 14(6).
Kroeger, K.D., Crooks, S., Moseman-Valtierra, S. and Tang, J. 2017. Restoring tides to reduce methane emissions in impounded wetlands: A new and potent Blue Carbon climate change intervention. Science Reports, 7, 11914.
Krumhansl, K.A. and Scheibling, R.E. 2011. Detrital production in Nova Scotian kelp beds: patterns and processes. Marine Ecology Progress Series, 421, 67-82.
Krumhansl, K.A. and Scheibling, R.E. 2012. Production and fate of kelp detritus. Marine Ecology Progress Series, 467, 281-302.
Ladd, C.J., Duggan‐Edwards, M.F., Bouma, T.J., Pagès, J.F. and Skov, M.W. 2019. Sediment supply explains long‐term and large‐scale patterns in salt marsh lateral expansion and erosion. Geophysical Research Letters, 46(20), 11178-11187.
Ladd, C.J.T. 2021. Review on processes and management of saltmarshes across Great Britain. Proceedings of the Geologists' Association, 132(3), 269-283.
Lancaster, J. (ed.), McCallum, S., Lowe A.C., Taylor, E., Chapman A. and Pomfret, J. 2014. Development of detailed ecological guidance to support the application of the Scottish MPA selection guidelines in Scotland’s seas. Scottish Natural Heritage Commissioned Report No. 491. Native oysters - supplementary document.
Lancaster, J., McCallum, S., Lowe A.C., Taylor, E., Chapman A. and Pomfret, J. 2014. Development of detailed ecological guidance to support the application of the Scottish MPA selection guidelines in Scotland’s seas. Scottish Natural Heritage Commissioned Report No. 491. Maerl beds - supplementary document.
Lebrato, M., Iglesias-Rodríguez, D., Feely, R. A., Greeley, D., Jones, D.O.B., Suarez-Bosche, N., Lampitt, R.S. , Cartes, J.E., Green, D.R.H. and Alker, B. 2010. Global contribution of echinoderms to the marine carbon cycle: CaCO3 budget and benthic compartments. Ecological Monographs, 80, 441-467.
Lee, H.Z., Davies, I.M., Baxter, J.M., Diele, K. and Sanderson, W.G. 2020. Missing the full story: First estimates of carbon deposition rates for the European flat oyster, Ostrea edulis. Aquatic Conservation: Marine and Freshwater Ecosystems, 30(11), 2076-2086.
Legge, O., Johnson, M., Hicks, N., Jickells, T., Diesing, M., Aldridge, J., Andrews, J., Artioli, Y., Bakker, D.C., Burrows, M.T. and Carr, N. 2020. Carbon on the northwest European shelf: Contemporary budget and future influences. Frontiers in Marine Science, 7, 143.
Lemasson, A.J., Fletcher, S., Hall-Spencer, J.M. and Knights, A.M. 2017. Linking the biological impacts of ocean acidification on oysters to changes in ecosystem services: a review. Journal of Experimental Marine Biology and Ecology, 492, 49-62.
Lima, M.A.C. 2020. Assessing the carbon sink potential and impacts of global change on intertidal seagrass meadows in central southern England. PhD thesis, University of Brighton.
Lindenbaum, C., Bennell, J.D., Rees, E.I.S., McClean, D., Cook, W., Wheeler, A.J. and Sanderson, W.G. 2008. Carbon on the northwest European shelf: Contemporary budget and future influences. Journal of the Marine Biological Association of the United Kingdom, 88, 133-141.
Lovelock, C. E., and Duarte, C.M. 2019. Dimensions of Blue Carbon and emerging perspectives. Biology Letters, 15: 20180781.
Loxton, R.G. 2014. Monitoring invertebrates by pitfall trapping after excavation of the surface vegetation in two slacks at Newborough Warren – Ynys Llanddwyn SSSI in 2013. NRW Evidence Report No: 22. Natural Resources Wales, Bangor.
Luisetti, T., Turner, R.K., Andrews, J.E., Jickells, T.D., Kröger, S., Diesing, M., Paltriguera, L., Johnson, M.T., Parker, E.R., Bakker, D.C. and Weston, K. 2019. Quantifying and valuing carbon flows and stores in coastal and shelf ecosystems in the UK. Ecosystem Services, 35, 67-76.
Lutz, M., Dunbar, R. and Caldeira, K. 2002. Regional variability in the vertical flux of particulate organic carbon in the ocean interior. Global biogeochemical cycles, 16(3), 11-1.
MacDonald, M.A., de Ruyck, C., Field, R.H., Bedford, A. and Bradbury, R.B. 2017. Benefits of coastal managed realignment for society: Evidence from ecosystem service assessments in two UK regions. Estuarine, Coastal and Shelf Science, 105609.
Mackenzie C.L., Kent F.E.A., Baxter J.M. and Porter J.S. 2018. Genetic analysis of horse mussel bed populations in Scotland. Scottish Natural Heritage Research Report No. 1000.
Macreadie, P.I., Fowler, A.M. and Booth, D.J. 2011. Rigs‐to‐reefs: will the deep sea benefit from artificial habitat? Frontiers in Ecology and the Environment, 9(8), 455-461.
Macreadie, P.I., Hughes, A.R. and Kimbro, D.L. 2013. Loss of ‘Blue Carbon’ from Coastal Salt Marshes Following Habitat Disturbance. PLoS One, 8(7).
Macreadie, P.I., Serrano, O., Maher, D.T., Duarte, C.M. and Beardall, J. 2017. Addressing calcium carbonate cycling in blue carbon accounting. Limnology and Oceanography Letters.
Macreadie, P.I., Anton, A., Raven, J.A., Beaumont, N., Connolly, R.M., Friess, D.A., Kelleway, J.J., Kennedy, H., Kuwae, T., Lavery, P.S., Lovelock, C.E., Smale, D.A., Apostolaki, E.T., Atwood, T.B., Baldock, J., Bianchi, T.S., Chmura, G.L., Eyre, B.D., Fourqurean, J.W., Hall-Spencer, J.M., Huxham, M., Hendriks, I.E., Krause-Jensen, D., Laffoley, D., Luisetti, T., Marbà, N., Masque, P., McGlathery, K.J., Megonigal, J.P., Murdiyarso, D., Russell, B.D., Santos, R., Serrano, O., Silliman, B.R., Watanabe, K. and Duarte, C.M. 2019. The future of Blue Carbon science. Nature Communications, 10(3998).
Mainwaring, K., Tillin, H.M. and Tyler-Walters, H. 2014. Assessing the sensitivity of blue mussel (Mytilus edulis) to pressures associated with human activities. Joint Nature Conservation Committee, JNCC Report No. 506.
Mair, J. M., Moore, C.G., Kingston, P.F. and Harries, D.B. 2000. A review of the status, ecology and conservation of horse mussel Modiolus modiolus beds in Scotland. Scottish Natural Heritage Commissioned Report F99PA08.
Mao, J., Burdett, H.L., McGill, R.A.R., Newton, J., Gulliver, P. and Kamenos, N.A. 2020. Carbon burial over the last four millennia is regulated by both climatic and land use change. Global Change Biology, 26, 2496-2504.
Marbà, N. and Duarte, C. 2009. Mediterranean warming triggers seagrass (Posidonia oceanica) shoot mortality. Global Change Biology, 16(8), 2366-2375.
Marine Scotland, 2019. Improving protection given to Priority Marine Features - Response to Scoping Consultation.
Marine Scotland, 2020. Scotland’s Marine Assessment 2020: Intertidal Seagrass.
Martin, S., Clavier, J., Chauvaud, L. and Thouzeau, G. 2007. Community metabolism in temperate maerl beds. I. Carbon and carbonate fluxes. Marine Ecology Progress Series, 335, 19-29.
Martin, S. and Hall-Spencer, J.M. 2017. Effects of ocean warming and acidification on rhodolith/maërl beds. In: Rhodolith/maërl beds: A global perspective. Springer, Cham, pp.55-85.
Maynard, C., McManus, J., Crawford, R.M.M. and Paterson, D. 2011. A comparison of short-term sediment deposition between natural and transplanted saltmarsh after saltmarsh restoration in the Eden Estuary (Scotland). Plant Ecology & Diversity, 4(1), 103-113.
Maynard ,C. 2020. Saltmarsh creation for natural flood defence in the Tay & Eden Estuaries and the Dornoch Firth: Final Report. University of St Andrews.
Mazik, K., Strong, J., Little, S., Bhatia, N., Mander, L., Barnard, S. and Elliott, M. 2015. A review of the recovery potential and influencing factors of relevance to the management of habitats and species within Marine Protected Areas around Scotland. Scottish Natural Heritage Commissioned Report No. 771.
Mazik, K., Strong, J., Little, S., Bhatia, N., Mander, L., Barnard, S. and Elliott, M. 2015. A review of the recovery potential and influencing factors of relevance to the management of habitats and species within Marine Protected Areas around Scotland. Scottish Natural Heritage Commissioned Report No. 771.
MCCIP, 2018. Climate change and marine conservation: Seagrass (Eds. Dawson, K., Kennedy, H., Leegwater, E. and Brazier, P.).
McKay, D.W. and Fowler, S.L. 1997. Review of the Exploitation of the Mussel, Mytilus edulis, in Scotland. Scottish Natural Heritage Review. No 68.
McLeod, E., Chmura, G., Bouillon, S., Salm, R., Björk, M., Duarte, C., Lovelock, C., Schlesinger, W. and Silliman, B. 2011. A blueprint for blue carbon: toward an improved understanding of the role of vegetated coastal habitats in sequestering CO2. Frontiers in Ecology and the Environment, 9(10), 552-560.
Migné, A., Davoult, D. and Gattuso, J. P. 1998. Calcium carbonate production of a dense population of the brittle star Ophiothrix fragilis (Echinodermata: Ophiuroidea): role in the carbon cycle of a temperate coastal ecosystem. Marine Ecology Progress Series, 173, 305-308.
Miller, L.C., Smeaton, C., Yang, H. and Austin, W.E.N. 2023. Carbon accumulation and storage across contrasting saltmarshes of Scotland. Estuarine, Coastal and Shelf Science.
MMO, 2019. Identifying sites suitable for marine habitat restoration or creation. A report produced for the Marine Management Organisation by ABPmer and AER. MMO Project No: 1135, February 2019, 93pp.
Moore, C.G., Saunders, G.R. and Harries, D.B. 1998. The status and ecology of reefs of Serpula vermicularis L.(Polychaeta: Serpulidae) in Scotland. Aquatic Conservation: Marine and Freshwater Ecosystems, 8(5), 645-656.
Moore, C.G., Bates, C.R., Mair, J.M., Saunders, G.R., Harries, D.B. and Lyndon, A.R. 2009. Mapping serpulid worm reefs (Polychaeta: Serpulidae) for conservation management. Aquatic Conservation: Marine and Freshwater Ecosystems, 19, 226-236.
Moore, C.G., Harries, D.B., Cook, R.L., Hirst, N.E., Saunders, G.R., Kent, F.E.A., Trigg, C. and Lyndon, A.R. 2013. The distribution and condition of selected MPA search features within Lochs Alsh, Duich, Creran and Fyne. Scottish Natural Heritage Commissioned Report No. 566.
Moore, C.G., Harries, D.B., James, B., Cook, R.L., Saunders, G.R., Tulbure, K.W., Harbour, R.P. and Kamphausen, L. 2018. The distribution and condition of flame shell beds and other Priority Marine Features in Loch Carron Marine Protected Area and adjacent waters. Scottish Natural Heritage Research Report No. 1038.
Moore, C.G., Harries, D.B., Tulbure, K.W., Cook, R.L., Saunders, G.R., Lyndon, A.R., Kamphausen, L. and James, B. 2020. The current status of serpulid reefs, horse mussel beds and flame shell beds in Loch Creran SAC and MPA. Scottish Natural Heritage Research Report No. 1156.
Moore, C.G. and Harries, D.B. 2020. Monitoring for the possible spread of Didemnum vexillum into the subtidal environment of Loch Creran. Scottish Natural Heritage Research Report No. 1155.
Morris, J.T. and Jensen, A. 1998. The carbon balance of grazed and non‐grazed Spartina anglica saltmarshes at Skallingen, Denmark. Journal of Ecology, 86(2), 229-242.
Morris, R., Reach, I., Duffy, M., Collins, T. and Leafe, R. 2004. On the loss of saltmarshes in south‐east England and the relationship with Nereis diversicolor. Journal of Applied Ecology, 41.
Muenzel, D. and Martino, S. 2018. Assessing the feasibility of carbon payments and Payments for Ecosystem Services to reduce livestock grazing pressure on saltmarshes. Journal of Environmental Management, 225, 46-61.
NatureScot, 2019. Conservation Objectives and Advice to Support Management – East Mingulay SAC.
Nellemann, C., Corcoran, E., Duarte, C.M., Valdés, L., De Young, C., Fonseca, L. and Grimsditch, G. 2009. Blue Carbon. A Rapid Response Assessment. United Nations Environment Programme. GRID-Arendal.
NimbleFins, 2019. Average Car Journeys in the UK: Car Mileage UK 2021.
Normand, S., Svenning, J. and Skov, F. 2007. National and European perspectives on climate change sensitivity of the habitats directive characteristic plant species. Journal for Nature Conservation, 15(1), 41-53.
Occhipinti-Ambrogi, A. 2007. Global change and marine communities: alien species and climate change. Marine pollution bulletin, 55(7-9), 342-352.
Orr, K.K. 2013. Predicting the ecosystem effects of harvesting beach-cast kelp for biofuel. PhD thesis, University of Aberdeen.
Ortega, A., Geraldi, N.R., Alam, I., Kamau, A.A., Acinas, S.G., Logares, R., Gasol, J.M., Massana, R., Krause-Jensen, D. and Duarte, C.M. 2019. Important contribution of macroalgae to oceanic carbon sequestration. Nature Geoscience, 12(9), 748-754.
Orth, R., Carruthers, T., Dennison, W., Duarte, C., Fourqurean, J., Heck, K., Hughes, A., Kendrick, G., Kenworthy, W., Olyarnik, S., Short, F., Waycott, M. and Williams, S. 2006. A Global Crisis for Seagrass Ecosystems. BioScience, 56(12), 987.
Orth, R.J., Lefcheck, J.S., McGlathery, K.S., Aoki, L., Luckenbach, M.W., Moore, K.A., Oreska, M.P., Snyder, R., Wilcox, D.J. and Lusk, B. 2020. Restoration of seagrass habitat leads to rapid recovery of coastal ecosystem services. Science advances, 6(41).
Pace, M.C., Bailey, D.M., Donnan, D.W., Narayanaswamy, B.E., Smith, H.J., Speirs, D.C., Turrell, W.R. and Heath, M.R. 2021. Modelling seabed sediment physical properties and organic matter content in the Firth of Clyde, Earth System Science Data, 13, 5847-5866.
Pakeman, R., Alexander, J., Beaton, J., Brooker, R., Cummins, R., Eastwood, A., Fielding, D., Fisher, J., Gore, S., Hewison, R., Hooper, R., Lennon, J., Mitchell, R., Moore, E., Nolan, A., Orford, K., Pemberton, C., Riach, D., Sim, D., Stockan, J., Trinder, C. and Lewis, R. 2015. Species composition of coastal dune vegetation in Scotland has proved resistant to climate change over a third of a century. Global Change Biology, 21(10), 3738-3747.
Paradis, S., Goñi, M., Masqué, P., Durán, R., Arjona‐Camas, M., Palanques, A. and Puig, P. 2021. Persistence of Biogeochemical Alterations of Deep‐Sea Sediments by Bottom Trawling. Geophysical Research Letters, 48(2).
Patterson, A. 2019. Do Rabbits (Oryctolagus cuniculus L.) increase Species Diversity through their Grazing and Burrowing Activities on Dune Grassland Habitats in North East Scotland? Kirkton, St Cyrus NNR.
Pembrokeshire Marine Special Area of Conservation, 2008. Agreed Management Scheme.
Perry, F. and Tyler-Walters, H. 2018. Maerl beds. In: Tyler-Walters H. and Hiscock K. (eds). Marine Life Information Network: Biology and Sensitivity Key Information Reviews. Plymouth: Marine Biological Association of the United Kingdom.
Pessarrodona, A., Moore, P.J., Sayer, M.D. and Smale, D.A. 2018. Carbon assimilation and transfer through kelp forests in the NE Atlantic is diminished under a warmer ocean climate. Global Change Biology, 24(9), 4386-4398.
Plassmann, K., Jones, M.L.M. and Edwards‐Jones, G. 2010. Effects of long‐term grazing management on sand dune vegetation of high conservation interest. Applied Vegetation Science, 13(1), 100-112.
Pogoda, B., Brown, J., Hancock, B., Preston, J., Pouvreau, S., Kamermans, P., Sanderson, W. and von Nordheim, H. 2019. The Native Oyster Restoration Alliance (NORA) and the Berlin Oyster Recommendation: bringing back a key ecosystem engineer by developing and supporting best practice in Europe. Aquatic Living Resources, 32, 3.
Porter, J.S., Austin, W.E.N., Burrows, M.T., Clarke, D., Davies, G., Kamenos, N., Riegel, S., Smeaton, C., Page, C. and Want, A., 2020. Blue carbon audit of Orkney waters. Scottish Marine and Freshwater Science, 11(3).
Potouroglou, M., Whitlock, D., Milatovic, L., MacKinnon, G., Kennedy, H., Diele, K. and Huxham, M. 2021. The sediment carbon stocks of intertidal seagrass meadows in Scotland, Estuarine, Coastal and Shelf Science, 258.
Preen, A. and Marsh, H. 1995. Response of dugongs to large-scale loss of seagrass from Hervey Bay, Queensland Australia. Wildlife Research, 22(4), 507.
Pye, K., Blott, S.J. and Howe, M.A. 2014. Coastal dune stabilization in Wales and requirements for rejuvenation. Journal of Coastal Conservation, 18, 27-54.
Queirós, A.M., Stephens, N., Widdicombe, S., et al. 2019. Connected macroalgal‐sediment systems: Blue carbon and food webs in the deep coastal ocean. Ecological Monographs, 89(3), e01366.
Ratcliffe, J.L., Payne, R.J., Sloan, T.J., Smith, B., Waldron, S., Mauquoy, D., Newton, A., Anderson, A.R., Henderson, A. and Andersen, R. 2018. Holocene carbon accumulation in the peatlands of northern Scotland. Mires and Peat, 23(3), 1-30.
Repolho, T., Duarte, B., Dionísio, G., Paula, J.R., Lopes, A.R., Rosa, I.C., Grilo, T.F., Caçador, I., Calado, R. and Rosa, R. 2017. Seagrass ecophysiological performance under ocean warming and acidification. Scientific Reports, 7(1), 1-12.
Roberts, J.M. 2002. The occurrence of the coral Lophelia pertusa and other conspicuous epifauna around an oil platform in the North Sea. Underwater Technology, 25(2), 83-92.
Roberts, J.M., Long, D., Wilson, J.B., Mortensen, P.B. and Gage, J.D. 2003. The cold-water coral Lophelia pertusa (Scleractinia) and enigmatic seabed mounds along the north-east Atlantic margin: are they related? Marine pollution bulletin, 46(1), 7-20.
Rodriguez-Perez, A., James, M., Donnan, D.W., Henry, T.B., Moller, L.F. and Sanderson, W.G. 2019. Conservation and restoration of a keystone species: Understanding the settlement preferences of the European oyster (Ostrea edulis). Marine Pollution Bulletin, 138, 312-321.
Rodriguez-Perez, A., Sanderson, W.G., Møller, L.F., Henry, T.B. and James, M. 2020. Return to sender: The influence of larval behaviour on the distribution and settlement of the European oyster Ostrea edulis. Aquatic Conservation: Marine Freshwater Ecosystems, 30(11), 2116-2132.
Röhr, M.E., Holmer, M., Baum, J.K., Björk, M., Boyer, K., Chin, D., Chalifour, L., Cimon, S., Cusson, M., Dahl, M. and Deyanova, D. 2018. Blue carbon storage capacity of temperate eelgrass (Zostera marina) meadows. Global Biogeochemical Cycles, 32(10), 1457-1475.
Ross, R.E. and Howell, K. L. 2013. Use of predictive habitat modelling to assess the distribution and extent of the current protection of ‘listed’ deep-sea habitats. Diversity and Distributions, 19(4), 433-445.
Rouse, S., Spencer Jones, M.E. and Porter, J.S. 2014. Spatial and temporal patterns of bryozoan distribution and diversity in the Scottish sea regions. Marine Ecology, 35, 85-102.
RSPB, 1995. Annual Report of the Royal Society for the Protection of Birds 1995. RSPB, Sandy, Bedfordshire.
Ruggiero, P., Hacker, S., Seabloom, E. and Zarnetske, P. 2018. The role of vegetation in determining dune morphology, exposure to sea-level rise, and storm-induced coastal hazards: a US Pacific Northwest perspective. In: Barrier dynamics and response to changing climate (pp. 337-361). Springer, Cham.
Sala, E., Mayorga, J., Bradley, D. et al. 2021. Protecting the global ocean for biodiversity, food and climate. Nature, 592, 397-402.
Santschi, P., Höhener, P., Benoit, G. and Buchholtz-ten Brink, M. 1990. Chemical processes at the sediment-water interface. Marine Chemistry, 30, 269-315.
Sanvicente Anorve, L.E. 1995. Détermination des structures benthiques spatiales en Manche Orientale au moyen de méthodes d'analyse multivariable et de techniques d'interpolation (These de Doctorat, Université Paris VI).
Sas, H., Deden, B., Kamermans, P., zu Ermgassen, P.S., Pogoda, B., Preston, J., Helmer, L., Holbrook, Z., Arzul, I., van der Have, T. and Villalba, A. 2020. Bonamia infection in native oysters (Ostrea edulis) in relation to European restoration projects. Aquatic conservation: marine and freshwater ecosystems, 30(11), 2150-2162.
Savini, A., Basso, D., Bracchi, V.A., Corselli, C. and Pennetta, M. 2012. Maerl-bed mapping and carbonate quantification on submerged terraces offshore the Cilento peninsula (Tyrrhenian Sea, Italy). Geodiversitas, 34(1), 77-98.
Sayer-Mitchell, J.D., Roberts, K.C., Schulz, P.H. and Brown, H. 2023. Loch Ailort serpulids: diving, ROV, and multibeam survey, 27 February – 3 March 2023. NatureScot Survey Report.
Scottish Government, 2023. Scottish Greenhouse Gas Statistics 2020.
Scottish Forestry, 2022. Diverse forests needed to cope with storm damage.
Simon-Nutbrown, C., Hollingsworth, P.M., Fernandes, T.F., Kamphausen, L., Baxter, J.M. and Burdett, H.L. 2020. Species Distribution Modelling Predicts Significant Declines in Coralline Algae Populations Under Projected Climate Change With Implications for Conservation Policy. Frontiers in Marine Science, 7, 758.
SMA, 2020b. Scotland’s Marine Assessment, 2020 - Case study: Persistent damage to the Loch Creran serpulid reefs.
Smale, D., Moore, P., Queirós, A., Higgs, N. and Burrows, M. 2018. Appreciating interconnectivity between habitats is key to blue carbon management. Frontiers in Ecology and the Environment, 16(2), 71-73.
Smale, D.A., Burrows, M.T., Moore, P., O'Connor, N. and Hawkins, S.J. 2013. Threats and knowledge gaps for ecosystem services provided by kelp forests: a northeast Atlantic perspective. Ecology and Evolution, 3(11), 4016-4038.
Smale, D.A., Pessarrodona, A., King, N., Burrows, M.T., Yunnie, A., Vance, T. and Moore, P. 2020. Environmental factors influencing primary productivity of the forest-forming kelp Laminaria hyperborea in the northeast Atlantic. Scientific Reports, 10(1), 1-12.
Smale, D.A., Pessarrodona A., King N. and Moore P.J. 2022. Examining the production, export, and immediate fate of kelp detritus on open-coast subtidal reefs in the Northeast Atlantic. Limnology and Oceanography, 67(S2), S36-S49.
Smeaton, C. and Austin, W.E. 2017. Sources, sinks, and subsidies: Terrestrial carbon storage in mid‐latitude fjords. Journal of Geophysical Research: Biogeosciences, 122(11), 2754-2768.
Smeaton, C. and Austin, W.E.N. 2019. Where’s the Carbon: Where’s the Carbon: Exploring the Spatial Heterogeneity of Sedimentary Carbon in Mid-Latitude Fjords. Frontiers in Earth Science, 7, 269.
Smeaton, C., Austin, W. and Turrell, W.R. 2020. Re-Evaluating Scotland’s Sedimentary Carbon Stocks. Scottish Marine and Freshwater Science, 11(2),16.
Smeaton, C., Austin, W.E., Davies, A., Baltzer, A., Howe, J.A. and Baxter, J.M. 2017. Scotland's forgotten carbon: a national assessment of mid-latitude fjord sedimentary carbon stocks. Biogeosciences, 14, 5663-5674.
Smeaton, C., Hunt, C.A., Turrell, W.R. and Austin, W.E.N. 2021a. Marine Sedimentary Carbon Stocks of the United Kingdom’s Exclusive Economic Zone. Frontiers in Earth Science, 9.
Smeaton, C. Yang, H. and Austin, W.E.N. 2021b. Carbon burial in the mid-latitude fjords of Scotland, Marine Geology, 441.
Smeaton, C. and Austin, W.E.N. 2022a. Quality not quantity: Prioritizing the management of sedimentary organic matter across continental shelf seas. Geophysical Research Letters, 49, e2021GL097481.
Smeaton, C., Burden, A., Ruranska, P., Ladd, C.J.T., Garbutt, A, Jones, L., McMahon, L., Miller, L.C., Skov, M.W. and Austin, W.E.N. 2022b. Using citizen science to estimate surficial Blue Carbon stocks in Great British saltmarshes. Frontiers in Marine Science, 9.
Smith, A.M., Key Jr, M.M. and Gordon, D.P., 2006. Skeletal mineralogy of bryozoans: taxonomic and temporal patterns. Earth-Science Reviews, 78(3-4), pp.287-306.
Smith, A.M., Riedi, M.A. and Winter, D.J. 2013. Temperate reefs in a changing ocean: skeletal carbonate mineralogy of serpulids. Marine Biology, 160, 2281-2294.
Smith, A.M. and Garden, C.J. 2013. Being a bimineralic bryozoan in an acidifying ocean. In: Bryozoan Studies 2010. Springer, Berlin, Heidelberg, pp.327-337.
Smith, A.M. 2014. Growth and calcification of marine bryozoans in a changing ocean. The Biological Bulletin, 226(3), 203-210.
Smith, A.M., Key Jr, M.M. and Gordon, D.P. 2006. Skeletal mineralogy of bryozoans: taxonomic and temporal patterns. Earth-Science Reviews, 78(3-4), 287-306.
Smith, R., Bianchi, T., Allison, M. et al. High rates of organic carbon burial in fjord sediments globally. Nature Geosci 8, 450–453 (2015).
Smout, T.C. and Stewart, M., 2012. The Firth of Forth – an Environmental History. Edinburgh: Birlinn Publications, 306pp.
SNH (Scottish Natural Heritage), 2017. Case study - Taking an adaptive approach: sea level rise and restoring the natural mobility of the Culbin sand dunes.
SNH (Scottish Natural Heritage), 2018a. Reviews of PMFs outside Scottish MPA network – Maerl beds.
SNH (Scottish Natural Heritage), 2018b. Reviews of PMFs outside Scottish MPA network - Serpulid aggregations.
SNH (Scottish Natural Heritage), 2018c. Reviews of PMFs outside Scottish MPA network - Blue mussel beds.
SNH (Scottish Natural Heritage), 2018d. Reviews of PMFs outside Scottish MPA network – Native oysters.
SNH (Scottish Natural Heritage), 2018e. Reviews of PMFs outside Scottish MPA network – Cold water coral reefs.
SNH (Scottish Natural Heritage), 2018f. Reviews of PMFs outside Scottish MPA network – Horse mussel beds.
SNH (Scottish Natural Heritage), 2018g. Reviews of PMFs outside Scottish MPA network – Flame shell beds
Strachan, I.M. 2017. Manual of terrestrial EUNIS habitats in Scotland. Version 2. Scottish Natural Heritage Commissioned Report No. 766.
Taylor, B.W., Paterson, D.M. and Baxter, J.M. 2019. Sediment Dynamics of Natural and Restored Bolboschoenus maritimus Saltmarsh. Frontiers in Ecology and Evolution, 7, 237.
The Inshore Fishing – Prohibition of Fishing and Fishing Methods (Scotland) Order 2015 (SI 2015/435).
Transport Scotland, 2019. Scottish Transport Statistics No. 38 2019 Edition.
Trendall, J., Bedford, G., Tarrant, D., Fortune, F. and Saunders, G. 2011. Baseline Survey and mapping of intertidal features within selected Scottish East Coast SSSI and Ramsar Sites. Scottish Natural Heritage Commissioned Report No. 447.
Trevathan-Tackett, S., Kelleway, J., Macreadie, P., Beardall, J., Ralph, P. and Bellgrove, A. 2015. Comparison of marine macrophytes for their contributions to blue carbon sequestration. Ecology, 96(11), 3043-3057.
Trigg, C. and Moore, C.G. 2009. Recovery of the biogenic nest habitat of Limaria hians (Mollusca: Limacea) following anthropogenic disturbance. Estuarine, Coastal and Shelf Science, 82, 351-356.
Tulbure, K.W. 2015. Investigating the condition of the Priority Marine Feature, ‘serpulid aggregations’ (Serpula vermicularis) in Loch Creran, Scotland. BSc(Hons) thesis, Heriot-Watt University.
Turrell, W.R., Austin, W.E.N, Philbrick, S.P., Tilbrook, C. and H. Kennedy (In Review). Clarifying the role of inorganic carbon in Blue Carbon policy and practice. Marine Policy.
UKCEH, 2021. UK Sand Dune and Shingle Network and Dynamic Dunescapes partners. The Sand Dune Managers Handbook. Version 1, June 2021. Produced for the Dynamic Dunescapes (DuneLIFE) project: LIFE17 NAT/UK/000570; HG-16-08643
University Marine Biological Station Millport, 2007. Conservation of the Native Oyster Ostrea edulis in Scotland. Scottish Natural Heritage Commissioned Report No. 251.
Unsworth, R.K., Bertelli, C., Cullen-Unsworth, L., Esteban, N., Lilley, R., Jones, B.L., Lowe, C., Nuuttila, H. and Rees, S. 2019. Sowing the seeds of seagrass recovery using hessian bags. Frontiers in Ecology and Evolution, 7, 311.
Van der Biest, K., De Nocker, L., Provoost, S., Boerema, A., Staes, J., Meire, P. 2017. Dune dynamics safeguard ecosystem services. Ocean & Coastal Management, 149, 148-158.
van der Heijden, L. and Kamenos, N. 2015. Reviews and syntheses: Calculating the global contribution of coralline algae to total carbon burial. Biogeosciences, 12(21), 6429-6441.
van Katwijk, M.M., Thorhaug, A., Marbà, N., Orth, R.J., Duarte, C.M., Kendrick, G.A., Althuizen, I.H.J., Balestri, E., Bernard, G., Cambridge, M.L., Cunha, A., Durance, C., Giesen, W., Han, Q., Hosokawa, S., Kiswara, W., Komatsu, T., Lardicci, C., Lee, K.‐S., Meinesz, A., Nakaoka, M., O'Brien, K.R., Paling, E.I., Pickerell, C., Ransijn, A.M.A. and Verduin, J.J. 2015. Global analysis of seagrass restoration: The importance of largescale planting. Journal of Applied Ecology, 53, 567-578.
Walker, F. 1954. Distribution of Laminariaceae around Scotland. Nature, 173(4408), 766-768.
Walker, F.T. and Richardson, W.D. 1955. An ecological investigation of Laminaria cloustoni edm. (L.hyperborea Fosl.) around Scotland. Journal of Ecology, 43, 26-38.
Walker, S.E. and Goldstein, S.T. 1999. Taphonomic tiering: experimental field taphonomy of molluscs and foraminifera above and below the sediment–water interface. Palaeogeography, Palaeoclimatology, Palaeoecology, 149, 227-244.
Waycott, M., Duarte, C., Carruthers, T., Orth, R., Dennison, W., Olyarnik, S., Calladine, A., Fourqurean, J., Heck, K., Hughes, A., Kendrick, G., Kenworthy, W., Short, F. and Williams, S. 2009. Accelerating loss of seagrasses across the globe threatens coastal ecosystems. Proceedings of the National Academy of Sciences, 106(30), 12377-12381.
Whitlock, D.S. 2021. Understanding the drivers of carbon sequestration in Scottish seagrass. PhD thesis, Edinburgh Napier University.
Wildish, D., Akagi, H.M. and Hamilton, N. 2000. Effect of Velocity on Horse Mussel Initial Feeding Behavior (No. 2325). St. Andrews, NB: Fisheries and Oceans Canada.
Wong, P.P., Losada, I.J., Gattuso, J.-P., Hinkel, J., Khattabi, A., McInnes, K.L., Saito, Y., Sallenger, A. 2014. Coastal systems and low-lying areas. In: Field, C.B., Barros, V.R., Dokken, D.J., Mach, K.J., Mastrandrea, M.D., Bilir, T.E., Chatterjee, M., Ebi, K.L., Estrada, Y.O., Genova, R.C., Girma, B., Kissel, E.S., Levy, A.N., MacCracken, S., Mastrandrea, P.R. and White, L.L. (eds). Climate Change 2014: Impacts, Adaptation, and Vulnerability. Part A: Global And Sectoral Aspects]. Contribution Of Working Group II to the Fifth Assessment Report Of the Intergovernmental Panel On Climate Change, pp. 361-409.
Wood, A.C.L., Probert, P.K., Rowden, A.A. and Smith, A.M. 2012. Complex habitat generated by marine bryozoans: a review of its distribution, structure, diversity, threats and conservation. Aquatic Conservation: Marine Freshwater Ecosystems, 22(4), 547-563.
Yan, J., Zhu, Z., Huang, H., Wang, X., Sui, H., Qiu, D., Bai, J. and Cui, B. 2019. Stage-dependent effects of a non-native invasive saltmarsh plant on the seedling establishment of a native seagrass. Ecohydrology & Hydrobiology, 20(4), 494-503.
Young, M.A., Macreadie, P.I., Duncan, C., Carnell, P.E., Nicholson, E., Serrano, O., Duarte, C.M., Shiell, G., Baldock, J. and Ierodiaconou, D. 2018. Optimal soil carbon sampling designs to achieve cost-effectiveness: a case study in blue carbon ecosystems. Biology letters, 14(9).
Yu, O.T. and Chmura, G.L. 2009. Soil carbon may be maintained under grazing in a St Lawrence Estuary tidal marsh. Environmental Conservation, 36(4), 312-320.
Yu, Z., Beilman, D.W. and Jones, M.C. 2009. Sensitivity of northern peatland carbon dynamics to Holocene climate change. pp.55-69. In: Carbon Cycling in Northern Peatlands.
Zhao, X., Guo, C., Han, Y., Che, Z., Wang, Y., Wang, X., Chai, X., Wu, H. and Liu, G. 2017. Ocean acidification decreases mussel byssal attachment strength and induces molecular byssal responses. Marine Ecology Progress Series, 565, 67-77.
Zimmerman, R.C., Hill, V.J., Jinuntuya, M., Celebi, B., Ruble, D., Smith, M., Cedeno, T. and Swingle, W.M. 2017. Experimental impacts of climate warming and ocean carbonation on eelgrass Zostera marina. Marine Ecology Progress Series, 566, 1-15.
Zu Ermgassen, P.S., Thurstan, R.H., Corrales, J., Alleway, H., Carranza, A., Dankers, N., DeAngelis, B., Hancock, B., Kent, F., McLeod, I. and Pogoda, B. 2020. The benefits of bivalve reef restoration: A global synthesis of underrepresented species. Aquatic Conservation: Marine and Freshwater Ecosystems, 30(11), 2050-2065.